New: https://www.mdpi.com/2075-1729/15/3/433
The Origin of Life in the Early Continental Crust: A Comprehensive Model
by Ulrich Schreiber
Life 2025, 15(3), 433; https://doi.org/10.3390/life15030433
Abstract
Continental rift zones on the early Earth provided essential conditions for the emergence of the first cells. These conditions included an abundant supply of raw materials, cyclic fluctuations in pressure and temperature over millions of years, and transitions of gases between supercritical and subcritical phases. While evidence supports vesicle formation and the chemical evolution of peptides, the mechanism by which information was stored remains unresolved. This study proposes a model illustrating how interactions among organic molecules may have enabled the encoding of amino acid sequences in RNA. The model highlights the interplay between three key molecular components: a proto-tRNA, the vesicle membrane, and short peptides. The vesicle membrane acted as a reservoir for hydrophobic amino acids and facilitated their attachment to proto-tRNA. As a single strand, proto-tRNA also served as proto-mRNA, enabling it to be read by charged tRNAs. By replicating this information and arranging RNA strands, the first functional peptides such as pore-forming proteins may have formed, thus improving the long-term stability of the vesicles. This model further outlines how these vesicles may have evolved into the earliest cells, with enzymes and larger RNA molecules giving rise to tRNA and ribosomal structures. Shearing forces may have facilitated the first cellular divisions, representing a pre-LUCA stage.
Keywords:
origin of life; hydrothermal biochemistry; information storage; continental crust model; supercritical fluids; pre-LUCA stage
Origin of life
Abstract:
The storage of biochemical information, which is a prerequisite for the development of the first cell, is an unsolved problem affecting all concepts of the origin of life. However, if the protected environment in the continental crust is taken into account, completely new possibilities emerge for identifying processes that may have been crucial for the formation of the first cell. Under this background, we can hypothesize that cellular life began, with a self-sustaining cycle of molecular reaction steps and information storage of peptide sequences in RNA in a crustal depth of approximately 1000 m. This cycle was made possible in an open system bound to gas-permeable tectonic fracture zones with a high proportion of CO2 and N2. The formation of peptides and vesicles in supercritical CO2 and the chemical evolution of peptides have already been proven for the upper continental crust. Other hypothetical considerations include the interactions of vesicles with catalytic peptides and the formation of a proto-tRNA. Individual hydrophobic amino acids accumulate in the vesicle membrane, and their position in the membrane depends on the degree of hydrophobicity. By attaching adenine to the acceptor arm of the proto-tRNA at the tip, it can presumably penetrate the membrane and be linked to an amino acid. The depth of penetration can be controlled by the hydrophobicity of the opposite anti-codon, with adenine always taking the middle position for the hydrothermally formed hydrophobic amino acids.
To get started:
From star gas to the first cell - The origin of life in the continental crust (in German) May 28, 2024
Public evening lecture as part of the lecture series "Discovering origins: A journey from the beginnings of the earth to the emergence of humanity" by Professor Dr. Ulrich Schreiber (Duisburg-Essen)
https://www.wiko-greifswald.de/mediathek/beitrag/n/aus-dem-sternengas-zur-ersten-zelle-der-ursprung-des-lebens-in-der-kontinentalen-kruste-196975/
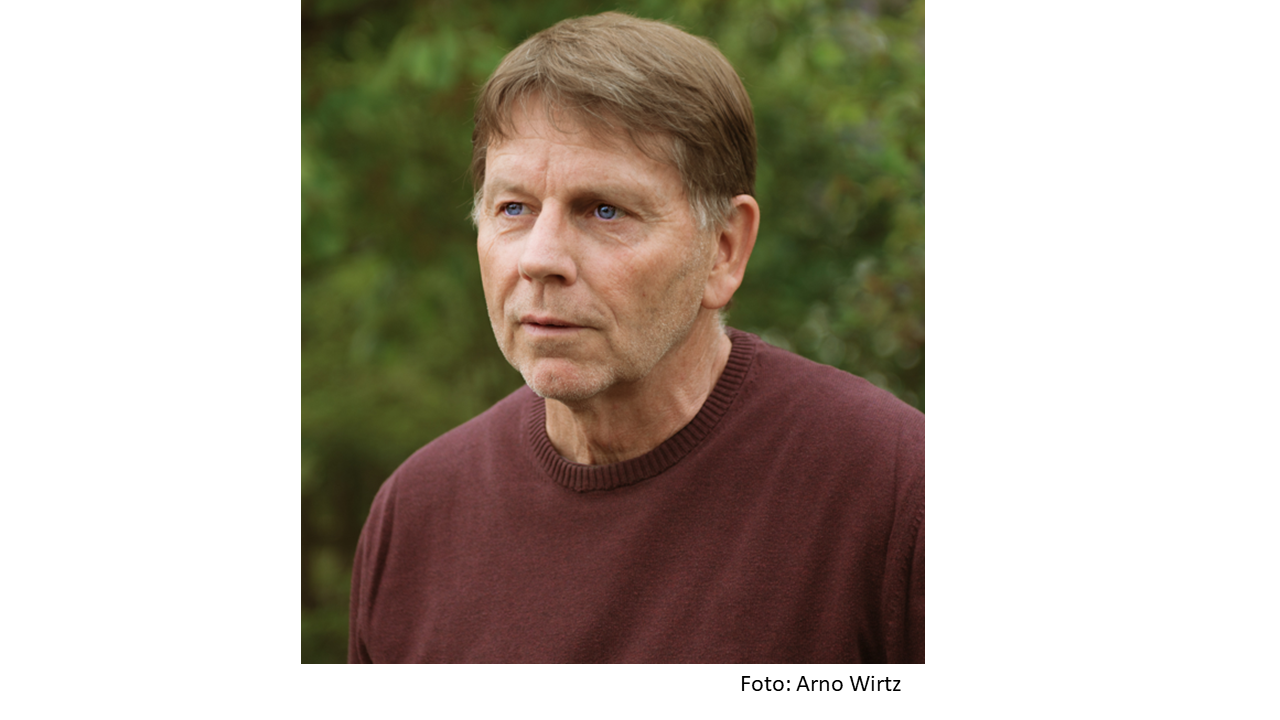
To investigate the cause of ebb and flow, it is not beneficial to merely observe the ocean.
The most precise examination of a rocket or an airplane provides no indication of the development of the earliest human tools, such as hand axes or knives. Yet, these tools form the foundation of the technological revolution.
How and where did life originate?
The continental crust as a model space for the formation of the first cell
Ulrich C. Schreiber; Bonn, November 2022
May 2024
Update: 25.08.2024; 14.09.2024; 6.01.2025
The all-encompassing question
How could an organic chemistry arise from inorganic substances, from gases, water and rocks, from which a biological information system developed? The storage of information about the blueprints of the cells, with all their biochemical instruments and coordinated reaction processes, represents the absolute basis of life. And how did life manage to assert itself against the ubiquitous increase in entropy and to build up orderly structures? These questions are among the greatest in science, apart from those concerning the actual origin of the universe.
At the University of Duisburg-Essen, together with the physical chemist Prof. Dr. Christian Mayer has developed a model in recent years that offers a scientific explanation for the first steps on the way to life [1]. The model is supported by experiments with a high-pressure cell (Fig. 1), which simulates realistic conditions as they still exist today. A more detailed understanding of the origin of the molecule, its concentration, questions of energy and entropy, and membrane formation has already been achieved [2].
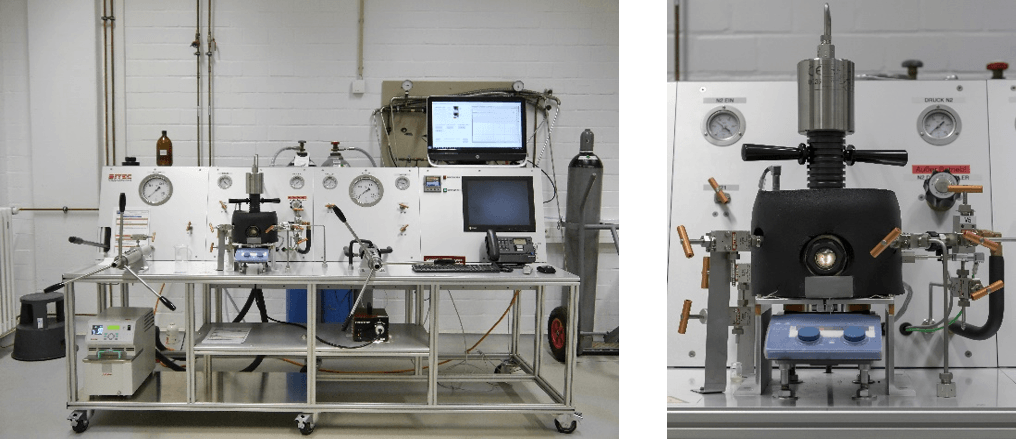
Fig. 1 left: high pressure system, right: detail with insulated pressure cell (50 ml)
What is it about?
The conditions of the young earth and the conditions for the emergence of life are largely in the dark due to the great temporal distance to today. A lack of boundary conditions prevents a clear delimitation of the possible processes, so that only narrowly limited statements on individual reactions could be made in a large number of precursor models. All localities on the earth's surface, from the deep sea to icy regions to shallow ponds, were discussed as possible environments. Due to criticism of the models and the lack of plausible alternatives, there have recently been proposals to include extraterrestrial regions such as Mars or meteorites in space as a whole.
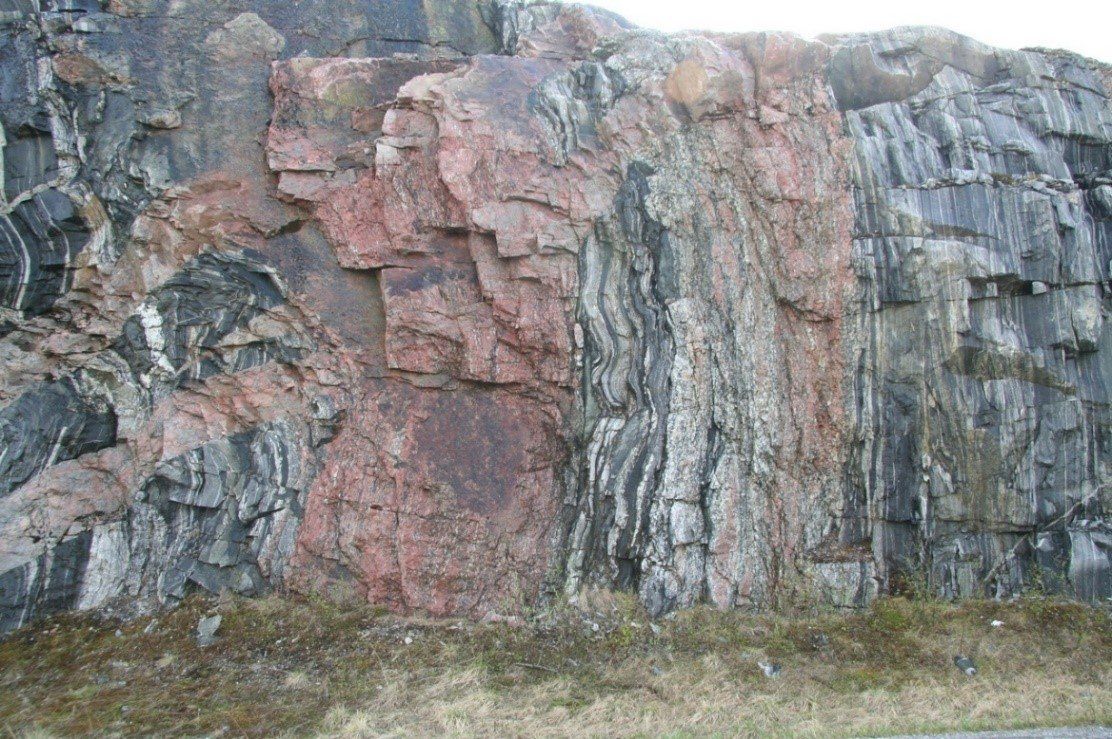
Fig. 2 Bedrock outcrop in northern Norway on the way to Kirkenes, near the border with Finland - Faults and fissures in the rock are filled by granitic magmas (pink veins) or hydrothermally formed quartz (white veins).
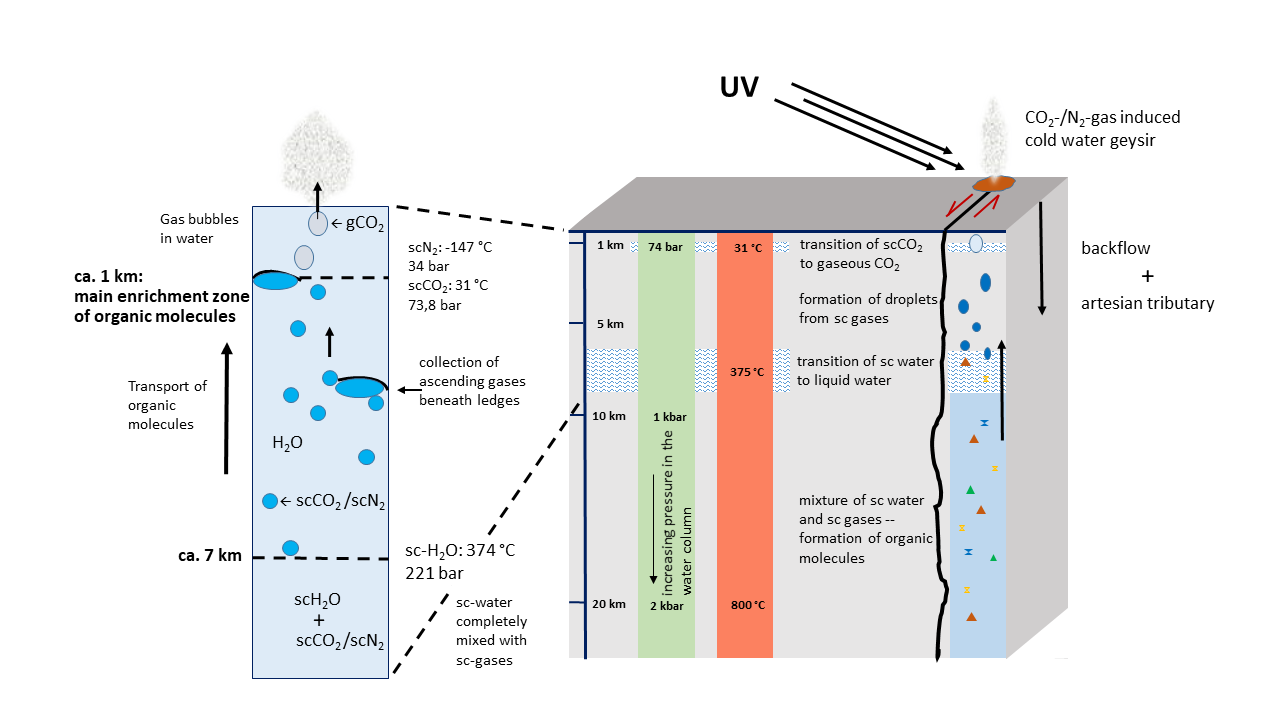
Fig. 3 Schematic Block Profile of Early Continental Crust. The average thickness of continental crust today is 30 km. Here, it is assumed to be lower. Simultaneously, the temperature must have been higher but could have been cooled in the upper portion by artesian waters.
The continental crust, which 4 billion years ago had grown to 25% of today's size [3], was only examined more closely by the Essen research group [1]. It differs from oceanic crust in that it is less dense and less warm, thicker, and has a more diverse mineralogical and chemical composition. If you look closely, the conditions within the continental crust are almost ideal for a start-up phase. By analyzing the framework conditions there, a large number of new approaches could be found that help to solve problems that are considered unsolvable in other models. The starting point is deep-reaching tectonic fault zones that are in contact with the earth's mantle. They are comparable to the San Andreas Fault or the Bavarian Pfahl fault in the Bavarian Forest, which is more than 200 km long and has been an active fault zone for more than 250 million years. Water, CO2 and other gases, which contain all the necessary substances for the formation of organic chemical molecules, rise from the depths, as is still the case today in the Eifel, for example.
One of the strongest arguments for examining this region more closely with regard to the origin of life is the CO2, which at a depth of approx. 800 meters undergoes a phase change from supercritical gas (scCO2, as it exists at depth) to gaseous CO2 (subcritical, gCO2, for the upper range) (critical point of the pure gas at 31 °C and 73.8 bar). In the supercritical state, scCO2 takes on the properties of both a liquid in which substances are dissolved and of a gas phase. Many chemical reactions can take place in scCO2 that are not possible in water. It acts like an organic solvent and greatly expands the number of possible chemical formation reactions. It also forms interfaces with water where special reactions can take place.
Due to a drop in pressure, the scCO2 changes to gCO2 in the border region from approx. 1000 m to 800 m depth (the pressure varies here due to the number of gas bubbles in the overlying water column). As a result, vesicles form with the formation of a double-layer membrane, which can be regarded as the precursor of the cell (see below). In addition to CO2, nitrogen (N2) is another gas that was pumped from the mantle into the atmosphere in large quantities and must have appeared in the upwelling channels of the fault zones in mixtures with CO2 or in pure form. The physical data (critical point of pure N2 gas at −146.9 °C and 33.96 bar) show that the supercritical state already occurs at about 350 m depth in an open water column (again depending on the number of gas bubbles in the supernatant water ). This has advantages for some chemical reactions, since the pH value is higher and more in the neutral range.
Basic steps on the way to a cell can already be documented in the laboratory [4]. This includes the formation of vesicles, which can be considered the first cell-like structures, and the linking of amino acids to form longer chains, which are the basis for the formation of complex molecules such as proteins and enzymes. What is particularly attractive for the model is the fact that the conditions for the emergence of life in hydrothermal vein rocks from the early days of the Earth have been partially documented. A large number of organic substances from this period are hidden in tiny liquid inclusions, such as those found by us in archaic vein quartz from Australia and from corresponding boulders in conglomerates (quartz crystallizes under hydrothermal conditions in fault zones) [5]. They were trapped and conserved billions of years ago during crystal formation. They help us to approximate the conditions for the laboratory tests to reality. The complex analysis was possible through the cooperation of the laboratories from Heidelberg (Heinz F. Schöler and Frank Keppler with Ines Mulder, Tobias Sattler and Markus Greule) and Essen (Christian Mayer with Maria Davila and Oliver J. Schmitz with Pia Rosendahl and Amela Bronja).
Fig. 4 Conglomerate in the Jack Hills, Western Australia, from which hydrothermal quartz boulders were analyzed. The oldest zircons to date have been found in the rock (> 4 billion years).
Even in almost recent mineral inclusions, the same series of molecules (aldehydes) can be found in the appropriate environment, which were formed inorganically from the gas components of the crust. For example, calcite could be extracted from a drill core from the CO2-carrying fissures of the young Wehr volcano (west of Lacher See) at a depth of almost 1000 m, which shows a comparable chemistry to archaic quartz from Australia. The analyzes were mainly carried out in cooperation with Prof. Oliver J. Schmitz in the chemistry department of the university [6].
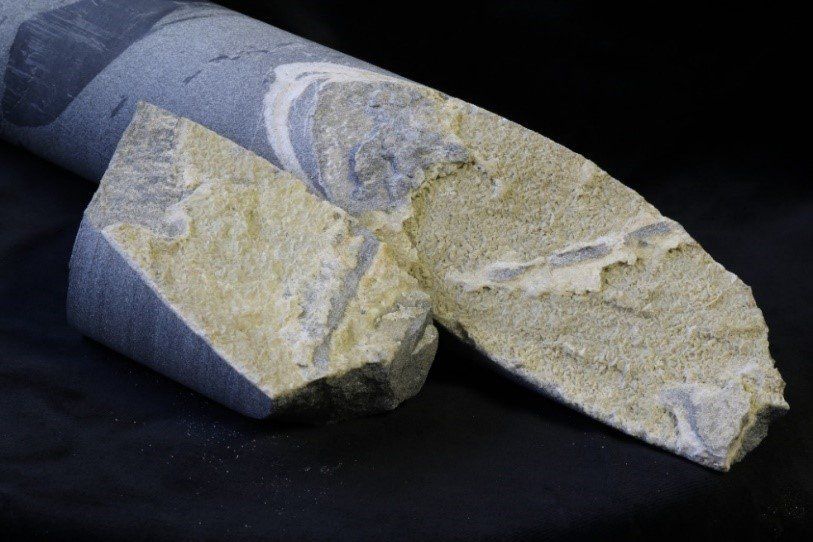
Fig. 5 Calcite-mineralized fissure in the drill core from the Wehrer Kessel (approx. 960 m depth), last eruption approx. 150,000 years ago, cracking in the rock due to volcanic tectonics, subsequently mineralized with calcite minerals, in which there are fluid inclusions with complex organic chemistry. Photo: Prof. Dr. O. J. Schmitz
However, research into the development of the biological information system in DNA or in the presumed precursor of RNA poses a particular challenge. The physicochemical conditions in the continental crust also appear to have been favorable for this process. The first signs of a solution are already emerging.
Fundamentals of the origin of life:
Since molecular tools such as enzymes did not exist at the beginning, the first steps towards life must have been purely physical or physicochemical. Thus, the formation of some of the components required for the cell can be explained by processes that are used daily in technical chemistry today. These include methods like the Fischer-Tropsch synthesis, which yields long-chain hydrocarbons, and the Haber-Bosch synthesis, producing ammonia (NH3) from N2 and H2. The requisite pressure-temperature conditions are present in the crust, along with the necessary raw materials. Furthermore, consideration must be given to the conditions and processes that were present or occurred overall, shaping the development of life within its emergence environment.
The Increase in Entropy as a Prerequisite for the Origin of Life:
- The linking of molecules, such as two amino acids, generates more order than previously existed in the system under consideration. Chemical reactions (e.g., condensation reactions) occur only if a higher degree of disorder is simultaneously formed, thus increasing entropy overall. For instance, heat may be generated in a test tube by a reaction, dissipating outwardly, thereby increasing entropy. Therefore, the increase in entropy (often described as an increase in disorder) must have been ensured throughout the course of life's development during reactions.
The transition from supercritical CO2 (sCO2) to gas (gCO2) at approximately 800 - 1000 m depth is associated with a significant increase in entropy.
- Typically, chemical reactions require energy to proceed, which must have been available to the necessary extent.
Heat energy, potential energy (from the rise of gases), and chemical energy (partially from piezoelectric voltage buildup due to seismic activity) were available in the crust. Additionally, there was a non-negligible radiation component due to the decay of radioactive elements in minerals.
- The environment had to remain stable over very long periods, protected from UV radiation, solar wind, weathering, meteorite impacts, and erosion. Hundreds of millions of years were necessary.
Fault zones such as the San Andreas Fault or the Bayerischer Pfahl attained this lifespan.
- The system had to remain open and out of equilibrium, allowing for the influx and efflux of substances. Molecules were consumed, but "unusable" molecules or reaction products could not clog the system (the problem of tar formation).
Water and molecules were transported toward the surface by rising gases in the faults.
- Cyclical changes in physical conditions must have prevailed. Cyclical conditions are more conducive to chemical evolution than sporadic events.
Cyclical pressure fluctuations occurred at the transition zone from scCO2 to gCO2 due to eruptions of cold-water geysers or significantly stronger Earth tides back then (the Moon was much closer to Earth), resulting in constant changes in conditions.
- Raw materials for the formation of organic molecules and necessary elements (metals, metalloids) had to be abundant.
Unlimited resources were available due to the influx of gases (CO2, N2, H2, H2S, etc.) from the mantle and the alteration of rocks, releasing phosphate and metals/metalloids.
- The formation of various organic molecules required different pressure-temperature (pT) conditions.
A transport system for bringing together the different molecular building blocks formed in various locations was one of the crucial requirements. Water and molecules formed at different depths were transported toward the surface by rising gases in the faults.
- Condensation reactions, such as the formation of a peptide from amino acids, can only occur if the water molecule produced during the reaction is removed.
In water (e.g., the cell), this is only possible with enzymes, thus in the early phase, a non-polar solvent is best suited. For example, in gas-rich fault zones, scCO2 or supercritical N2 serves as a non-polar solvent.
- Inorganic catalysts were likely required for the formation of RNA (tRNA) as the first carriers of information. Clay minerals have been discussed as potential catalysts based on laboratory studies.
Clay minerals form not only through weathering of surface rocks but also through alteration of rock-forming minerals in the Earth's crust by acidic waters in the faults.
- A fundamental problem on early Earth was the formation of a large number of different organic molecules, including in fault zones of the continental crust. There was essentially a molecular chaos from which specific molecules could not yet be filtered out by "biochemical tools." Only physical or physicochemical processes were available for pre-sorting:
Flow processes in the water-filled faults of the continental crust due to rising scCO2 gases (or gases in the uppermost 800 meters).
Surface effects allowing for the attachment of specific molecules to changing mineral surfaces (rock-forming minerals, vein ores such as lead-zinc or iron-sulfide ores, quartz, clay minerals) during ascent.
Flotation by scCO2/scN2 bubbles, which directly captured non-polar molecules or deposited certain amphiphiles on the bubble surface, acting as collector molecules for specific RNA molecules, for instance.
In the transition zone between 1000 m to approximately 800 m, there is a phase change of gases from supercritical to subcritical (gaseous) state (at lower depths for pure N2 systems). Due to pressure fluctuations, e.g., during cold-water geyser eruptions (CO2/N2-controlled eruptions), the depth of the transition to the subcritical gas phase varies over several tens of meters. The consequence is the precipitation of organic molecules because the forming subcritical gas can no longer hold dissolved molecules. This results in an enrichment horizon where concentrations of substances become high enough to favor chemical reactions.
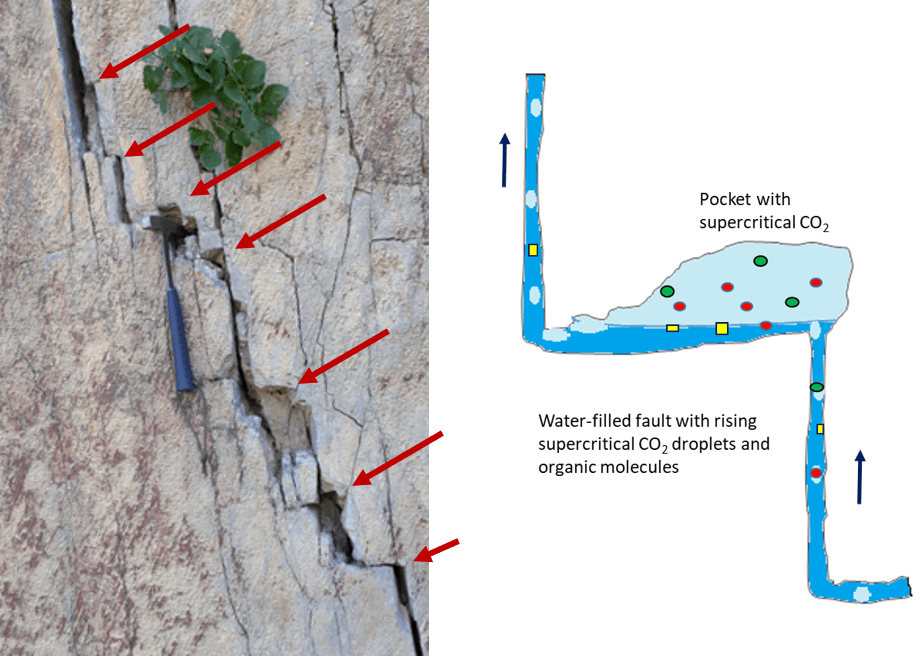
Fig. 6 Example of the conditions in a fault. The arrows point to traps for rising gases in case the system is filled with water in the crust (left - photo: Dr. Frederik Kirst). At greater depths below about 800 m, scCO2 collects under the ledges. Here, non-polar molecules can be enriched in the supercritical gas and react with each other (sketch on the right).
RNA
The formation of an RNA (as a precursor to DNA) is one of the most important prerequisites for the development of an organic-chemical information system. The conditions in the fault zones with the provision of organic bases, the sugar ribose and phosphate from the dissolution of the apatite were favorable for this [9]. However, not only the molecules that are present in today's RNA were formed in this environment. There was certainly a multitude of different sugars, which also appeared in the chiral D and L versions. Alteration of the crustal rocks resulted in clay mineral lining at various sections of the fault surfaces. They presumably acted as catalysts that controlled the linking of nucleotides to form longer RNAs [8]. These RNAs could be very different due to different sugars or bases. It is therefore necessary to identify processes which, without the help of today's biochemical catalysts, led to the RNA type that determined further events on the way to the first cell.
When longer RNA strands are formed, entanglement quickly occurs because complementary sections combine and sometimes form double-stranded sections [10]. A copy that could take place through the addition of free nucleotides is thus prevented, a core problem in the RNA world. By increasing the temperature, however, double strands are melted and are then available for doubling when they cool down. The conditions in the disturbances offer optimal conditions for the process of RNA copying. The temperature at a depth of 1000 m could have been slightly above 50 °C due to the inflow of surface water. Due to the transition of the scCO2 to the gas phase as a result of a geyser eruption, cooling is caused by the expansion of the gas (Joule-Thompson effect).
The reverse process compresses the gas, which is associated with an increase in temperature. Here, values well above 60 °C can be reached, which are necessary to separate RNA double strands. Additional temperature changes result from hotter water flowing in from the depths and then returning cooler after the end of the eruption. The phase of the attachment of complementary nucleotides begins with the cooling. If enough building blocks are available, RNA strands can be copied with each burst cycle. The same processes can take place with supercritical nitrogen (scN2) at shallower depths and correspondingly cooler background temperatures.
If the conditions in an environment are suitable for RNA formation, the basis for the development of an information system is not automatically given. Strands of RNA that form vary in length and are rapidly broken down into short sections by hydrolysis. The hydrolysis of an RNA strand is lowest in an acidic environment at pH values between 3 and 4, as is also the case in the fluids of the deep faults [11], i.e. the greatest range of stability is found here. There remains the molecular chaos that requires sorting.
Above a certain length, there is a high probability that there are sections with complementary bases in the first and last third of the RNA strand. This means that when there is a bend, the bases of both thirds are opposite and form a connection via base pairing (adenine A connects to uracil U, cytosine C to guanine G), as in a DNA double strand. A shorter version of a proto-tRNA could consist of only 12 nucleobases, with only one base pairing after the loop. The middle third forms a loop, in the bend of which three of the bases point outwards (by rotating within the strand) at a certain diameter of the sector of a circle, so that complementary bases can also attach themselves. This is a prototype of a tRNA (transfer RNA), which in today's cells, although much more complex, forms the mediator between a specific amino acid and an information code.
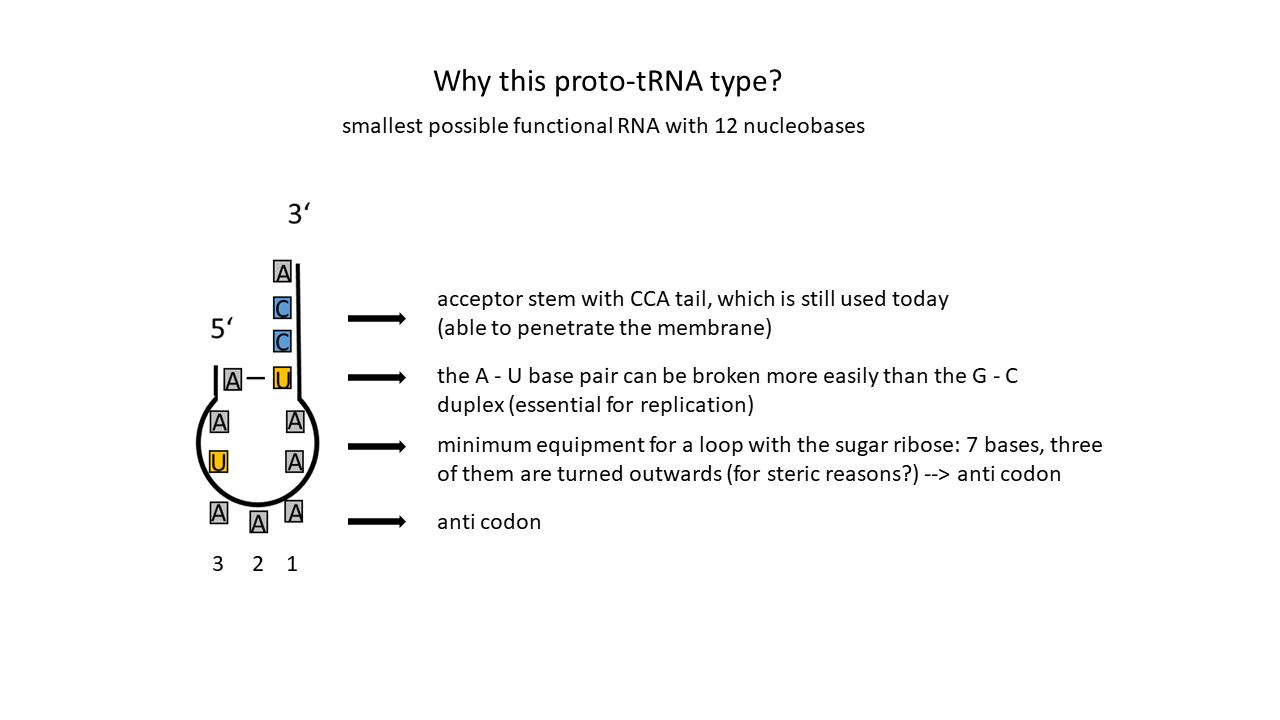
Fig. 7 Possible Proto-tRNA: Shortest possible RNA strand forming only one base pair and an extended single strand, with its last three bases always being C, C, A (Cytosine, Cytosine, Adenine). Three bases are positioned in the loop (here AAU (U = Uracil) in the anticodon; an outward-facing position requires a rotation within the strand), which can be complemented by complementary free bases. These form the codon, a triplet code composed of four different RNA bases that currently constitute RNA.
This work is licensed under a Creative Commons Attribution-NonCommercial-ShareAlike 4.0 International License.
The immediate question arises as to why ribose, a pentose sugar with 5 carbon atoms, is utilized in building the RNA strand instead of other pentoses or even hexoses, which form molecules with 6 carbon atoms. Such alternative nucleotide chains can be synthesized in the laboratory. Ultimately, the various sugars must have been present in a multitude of environments since their formation is not selectively restricted to just one type. Consequently, there likely existed a range of other types of RNA strands that had incorporated different sugars, all of which were also chiral and thus possessed two distinct handednesses.
What selection processes might have led to the choice of ribose? Certainly, stability criteria or simply the abundance of ribose must be considered. However, another crucial factor could be at play. When an RNA strand bends, all bases must be oriented inwards. This would function in a very long strand allowing for a large loop diameter. However, longer RNA strands are rare and are quickly hydrolyzed. If they do persist, they do not form a prototype of a tRNA suitable for information storage, as they lack the outward-facing information unit, the present-day anticodon. In shorter RNAs, which could form a loop with the minimum possible diameter, the inward-facing bases encounter spatial constraints. This problem can be resolved by twisting the strand in a section of the bend, thereby orienting the bases outward. Now, it must be compared whether the alternative sugars, both other pentoses and hexoses, can result in a configuration of the RNA loop that includes an outward-facing "readable" triplet. An arrangement of bases that impairs or makes readability impossible does not persist (as no information about enzymatically supportive catalysts is retained in further development). If this consideration is confirmed, ribose would have been selected from the multitude of sugars, in combination with the bases and phosphate, purely for steric reasons (structurally best suited to form an anticodon).
It is noteworthy that all tRNAs that are specifically loaded with an amino acid today always possess the same coupling strand of the base sequence CCA. This binding sequence must have proven to be so successful in the earliest phase that it continues to be exclusively utilized today. The synthetases, the complex enzymes responsible for the specific loading onto the corresponding tRNAs, recognize the now more complexly constructed tRNAs based on their additional loops and the specific bases contained therein, ensuring the very important precise assignment of the amino acid to be linked. The question remains as to why the version with the acceptor arm CCA emerged as presumably the sole representative from the group of RNAs of medium length in the selection process.
The cause may have lain in an advantage conferred by a specific property of the proto-tRNA. In principle, any amino acid could have bound to any proto-tRNA. Where was the possibility of a specific assignment, which was necessary for the construction of an information store? Present circumstances allow at least a rough differentiation. There are two classes of synthetases that carry out the specific loading of tRNAs. Class I synthetase catalyzes hydrophobic amino acids at the 2'-OH end of the terminal ribose, and class II synthetase catalyzes hydrophilic amino acids at the 3'-OH end.
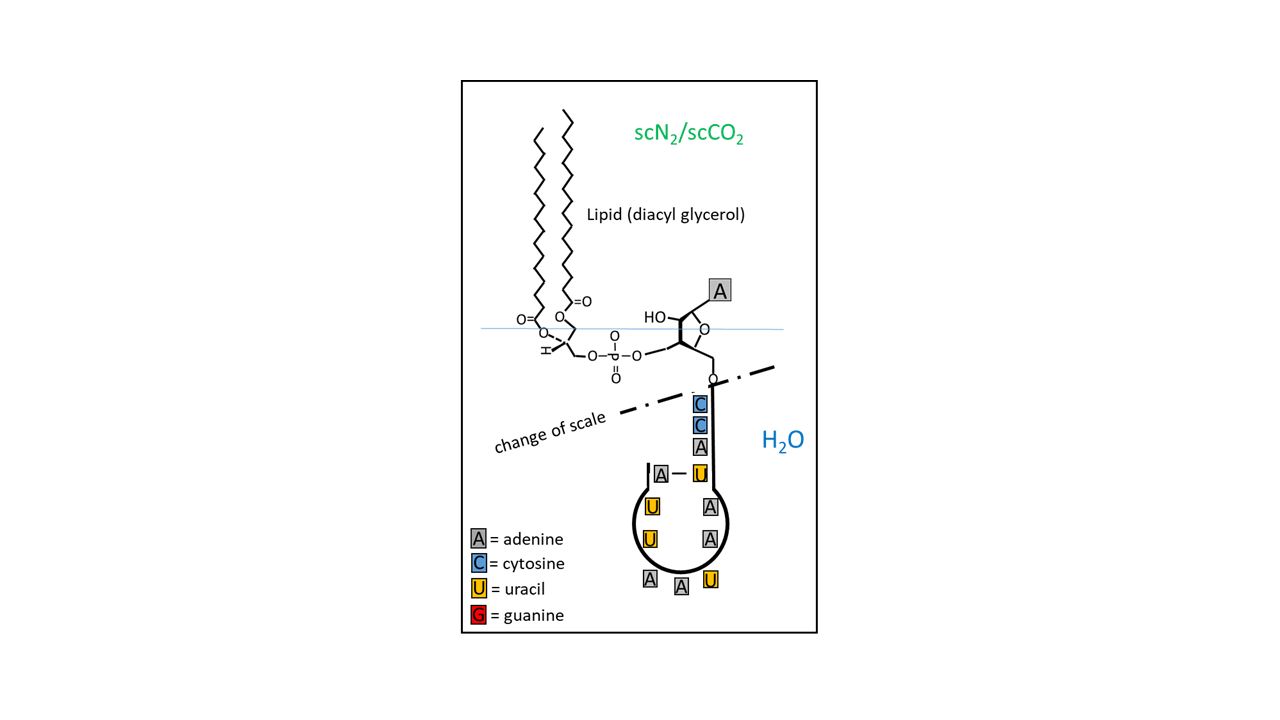
Fig. 8 Amphiphilic lipids are linked to the acceptor arm of a Proto-tRNA. Alternatively, hydrophobic amino acids can bind to the 2'-OH of ribose, and hydrophilic amino acids can bind to 3'-OH. The blue line, as a separation interface between a hydrophobic (above) and a hydrophilic environment (area distorted in scale), could serve as a possible demarcation line for the two linkage sites.
This work is licensed under a Creative Commons Attribution-NonCommercial-ShareAlike 4.0 International License.
However, it is necessary to first identify the enrichment potential of a specific type of RNA that possessed the characteristics of a proto-tRNA. It is possible that flotation processes, aided by collector molecules and ascending supercritical gas bubbles, transported suitable RNA molecules to the precipitation zone. Lipids (amphiphiles) are capable of this process, consisting of a hydrophilic head and a hydrophobic tail (see Fig. 8 and 9). The head can bind to an RNA molecule (in the case of a proto-tRNA, with the CCA end or other attachment sites) (see also [14]). While the hydrophobic portion can be encapsulated in a supercritical gas bubble, the hydrophilic remainder remains with the RNA in the water. The gas bubbles rise and transport the molecules to gas pockets, where they accumulate. From there or directly, they reach the boundary zone where the supercritical state transitions to the subcritical state. While other RNA variants can be transported similarly, hydrophobic organic molecules transfer directly into the bubbles and ascend with them. At approximately 1000 meters depth, during a phase transition caused by pressure decrease during a geyser eruption, all transported molecules are enriched in the water and at the interface with the gas phase.
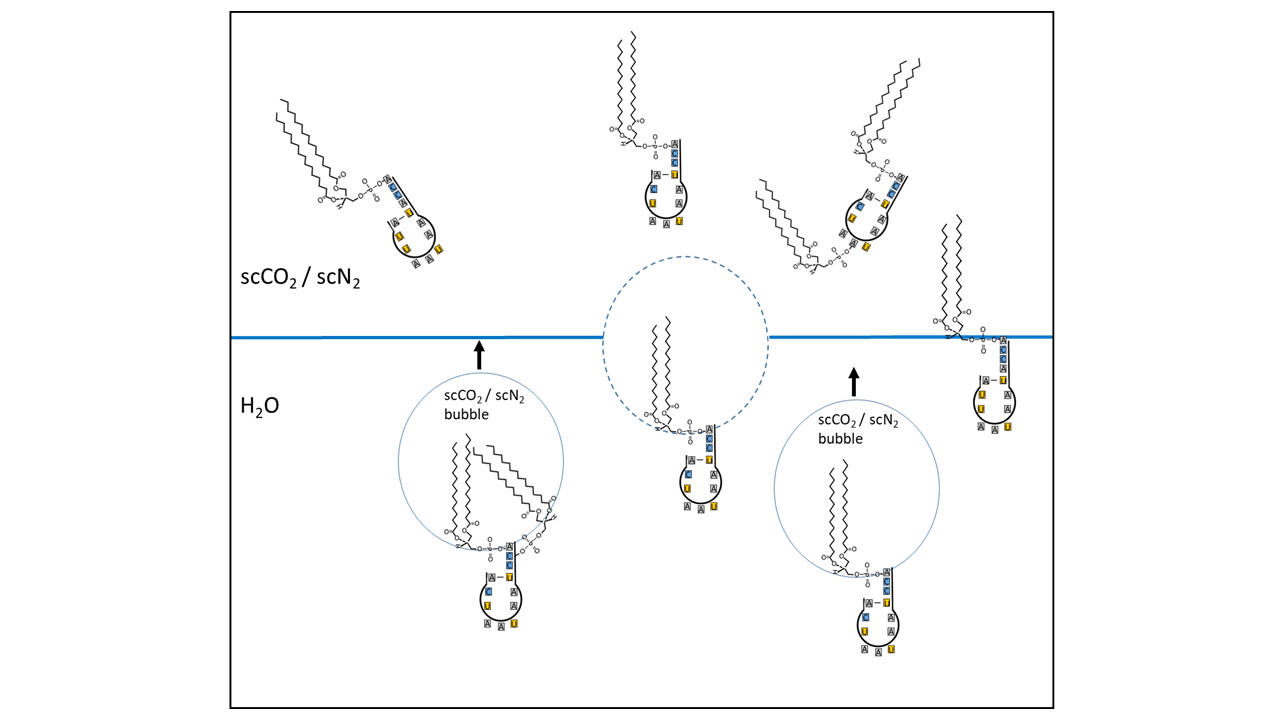
Fig. 9 Principle sketch of a flotation process: Amphiphilic lipids are linked to the acceptor arm of a proto-tRNA. The hydrophobic part is integrated into bubbles of supercritical CO2/N2, while the hydrophilic head with the RNA remains in the water. The bubbles rise and collect in the roof area of a cavity. Some of the molecules get completely into the scCO2/N2 reservoir, others position themselves exactly on the water surface. Other types of RNA are linked and transported in a similar way. Hydrophobic organic molecules can pass directly into the bubbles and rise with them. At a depth of around 1000 meters, with the phase change due to a decrease in pressure, all transported molecules in the water and at the interface with the gas are enriched.
This work is licensed under a
Creative Commons Attribution-NonCommercial-ShareAlike 4.0 International License.
Molecule separation, as in technical chromatographic processes
In the fissures of fault zones, fluid flows provide all the necessary conditions for separation processes of mixtures of organic molecules. The conditions resemble chromatographic analytical methods, where separations of various molecules occur through a dynamic interplay between solvent and solid phase. The cracks and fracture surfaces in the continental crust have cross-sectional sizes ranging from capillary dimensions to the order of several centimeters. These dimensions are comparable to those used in technical separation processes. Particularly interesting is the separation process when the state of the mobile phase is supercritical. Corresponding effects in the continental crust are directly comparable to supercritical fluid chromatography (SFC), which is predominantly performed with supercritical CO2. In this method, silica gels are used as the solid phase. Silica gels are typical of deeper-reaching faults, from which the gangue mineral quartz is formed, which typically lines the fracture surfaces and constitutes the basis for quartz veins. There are also methods such as the simulated moving bed (SMB) process, in which the solid phase flows counter to the substances to be separated. This would be the case if silica gels were transported upwards in the fluid flow of the faults. SMB processes are used, for example, in the pharmaceutical industry to separate molecules with different chirality in large quantities. The method is also employed for the industrial-scale purification of fructose. This could explain the use of ribose in RNA formation within the hydrothermal fissure systems of the crust. Through chromatographic separation of various sugars, ribose would have been most strongly enriched in this case.
The vesicle formation
Mayer et al. [2, 12] were able to demonstrate the formation of vesicles and chemical evolution of peptides in high-pressure experiments. The conditions of the upper crust were simulated with numerous cavities and a cold water geyser system. The focus of the experiments was on the depth of about 1000 m, the transition area from scCO2 to gas. A large number of cavities, in which the CO2 accumulates, appear along the tear-off edges on the fault surfaces. This creates reaction chambers with two phases, water in the lower part and scCO2 or gCO2 in the upper part. If scCO2 is present, the pressure drop during a geyser eruption in the border region leads to a phase change. CO2 gas is produced in which dissolved water condenses to form a mist (corresponds to salt-free condensed water).
V1 Video showing the interior of the high-pressure cell depicted in Figure 1: The lower half contains water, which is being stirred by a magnetic stirrer. The upper half contains supercritical scCO2 (scCO2) and gaseous CO2 (gCO2).
The pressure, initially above the threshold for supercritical CO2, is gradually reduced. As the pressure drops below the supercritical state, mist droplets form from the small amount of water dissolved in the scCO2. These droplets acquire an initial lipid coating within the gas phase. Upon descending to the water surface, which is also coated with lipids, a second lipid layer forms around the droplets. The result is vesicles composed of condensed water droplets enclosed by a lipid membrane.
As the experiments have shown, this is the starting point for vesicle formation, since lipids from the scCO2, which cannot remain in the gas, collect to form a first shell on the outer surface of the droplets. The sinking to the water interface, which is also covered with lipids, leads to a further encapsulation, so that vesicles with a double membrane are then present in the water. Added amino acids linked to form peptides during depressurization and interacted with the membrane of the formed vesicles.
V2 MD simulation as an example of the behavior of hydrophobic peptides (here 8 amino acids) in a membrane. The blue dotted areas are water molecules. The membrane consists of lipids with gray, hydrophilic heads and green, hydrophobic tails that point inwards. Clip courtesy of Maria Davila Garvin and Christian Mayer, Physical Chemistry at the University of Duisburg-Essen. Questions and suggestions should be directed to them directly: maria.davila@uni-due.de christian.mayer@uni-due.de
With a renewed increase in pressure due to back-flowing water after the end of the eruption, most of the vesicles are destroyed again. In the end, Mayer et al. show that chemical evolution is possible through cyclic repetition of the pressure fluctuations, which leads to the mutual stabilization of peptides and vesicles.
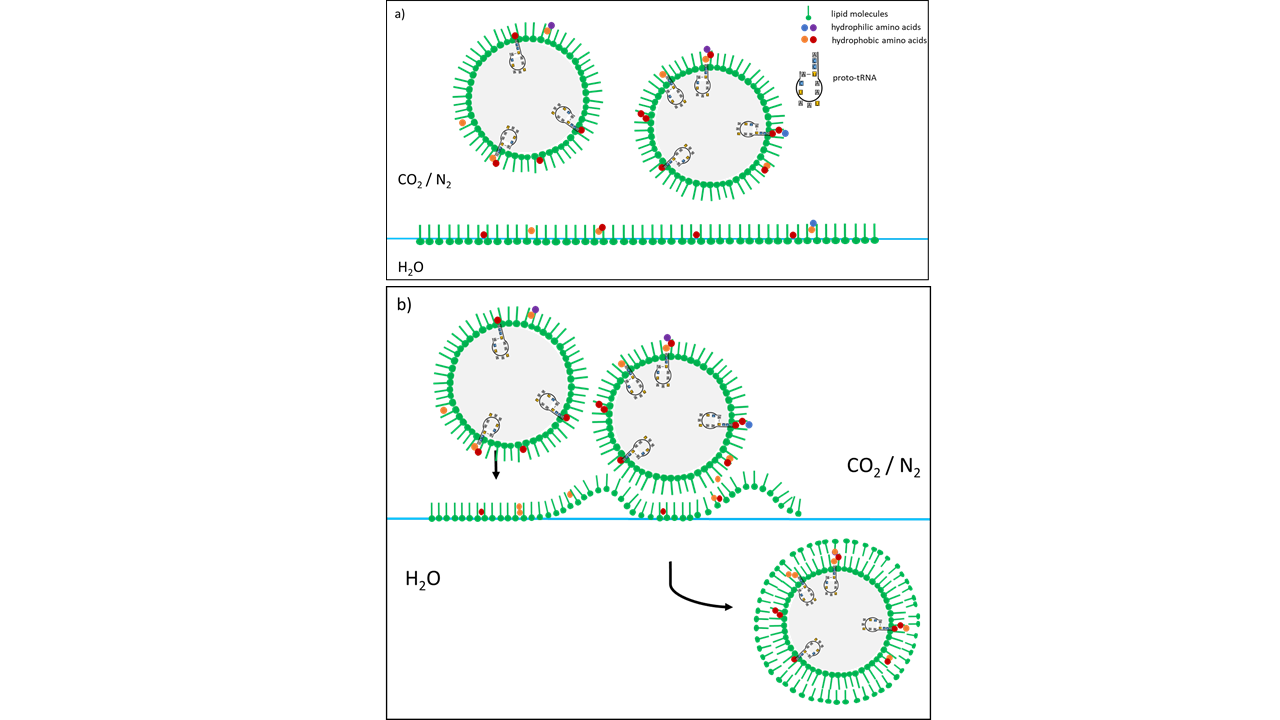
Fig. 10 Vesicle formation after depressurization resulting from a cold-water geyser eruption. a) Mist droplets are coated with a shell of lipids. b) In contact with lipids on the water surface, a second coating is formed (based on Mayer et al. 2015 [2]). The vesicles consist of distilled water with a proportion of organic molecules. The difference in salt concentrations inside and outside creates instability, leading to rapid destruction during the next geyser eruption. The same vesicle formation process occurs with water splashes that are thrown over the surface of the water during a turbulent geyser eruption. The vesicles that form are significantly larger, have a significantly higher concentration of organic molecules, but have the same salt content as the surrounding water. This means that they are more stable than the vesicles from the mist droplets and have higher concentrations of organic molecules for reactions.
This work is licensed under a
Creative Commons Attribution-NonCommercial-ShareAlike 4.0 International License.
However, given the seemingly infinite number of possible combinations in peptide formation, no repetition of the same sequences in the amino acid chains can be expected. What is missing is a storage of the information about the sequence, as it can be found in the later formed RNA or DNA.
However, the importance of vesicle formation is reflected in several properties that have played an important role in the development of life.
- On the one hand, it becomes clear how easily vesicles can be formed as precursors of cell compartments.
- On the other hand, an interaction between the membrane and molecules occurs, which can lead to special reactions and chemical evolution.
- Furthermore, the type of vesicle formation is suitable for taking up large molecular complexes that are formed in the free environment of the cavities during droplet formation.
January 2025
The results obtained so far give rise to far-reaching considerations for new research questions and experimental designs. For example, in addition to the canonical L-amino acids previously used, D-amino acids can be employed as alternatives in the same experimental series. It is of particular interest to investigate whether chemical selection in peptides containing D-amino acids leads to the same sequences as those observed in peptides with L-amino acids. Another experimental series could be conducted using racemic mixtures, where the proportions of both isomers are equal.
Of particular interest are the sequences of D-peptides for pharmaceutical research, especially in the context of developing new antibiotics. For this purpose, additional components must be integrated into the vesicle formation process to enable specific selective steps.
A possible experimental design involving D-amino acids and L-ribose in RNA could serve as a starting point for the development of a mirror-image cell.
Confronting risks of mirror life (DOI: 10.1126/science.ads9158)
The Hypothetical Model for Information Storage
For the storage of information regarding amino acid sequences in peptides, an intermediary molecule was required, which must have corresponded to a simplified version of a tRNA (as mentioned above). This proto-tRNA is assumed for the development of the first cell. The use of DNA as the carrier of genetic information is presumed to occur at a later stage (its stability under the conditions of hydrothermal disruption zones would not be assured).
The Onset of Information Storage
The formation of vesicles under pressure and temperature conditions resembling those at approximately 1000 meters depth in a continental crust of the young Earth (as mentioned above) has been demonstrated multiple times in laboratory settings [2, 4]. Up to 12 amino acids can arise under hydrothermal conditions in the crust [15, 16], with frequencies varying depending on the formation conditions. The (hydrothermally producible) hydrophobic amino acids isoleucine, valine, leucine, and phenylalanine can be incorporated into the membrane during vesicle formation, while the hydrophilic amino acids threonine, serine, proline, glutamic acid, aspartic acid, and lysine remain in the water inside the droplets. The vesicles consist of distilled water with a proportion of organic molecules. The difference in salt concentrations inside and outside leads to instability, resulting in rapid destruction during the next geyser eruption. The same vesicle formation process occurs with water sprays, which are thrown over the water surface of the cavity during a turbulent geyser eruption at depth. The resulting vesicles are significantly larger, possess a much higher concentration of organic molecules, but have the same salt content as the surrounding water. As a result, they are more stable than the vesicles from mist droplets and have higher concentrations of organic molecules for reactions.
In the simple proto-tRNA sketched above (Fig. 8), the base adenine with the base sequence CCA is positioned exactly at the tip of the unpaired strand. The acceptor arm can penetrate the membrane due to the hydrophobicity of the adenine and thus comes into contact with the hydrophobic amino acids (Fig. 11). A linkage at the 2'-OH position of the terminal ribose in the context of the geyser cycle is now possible.
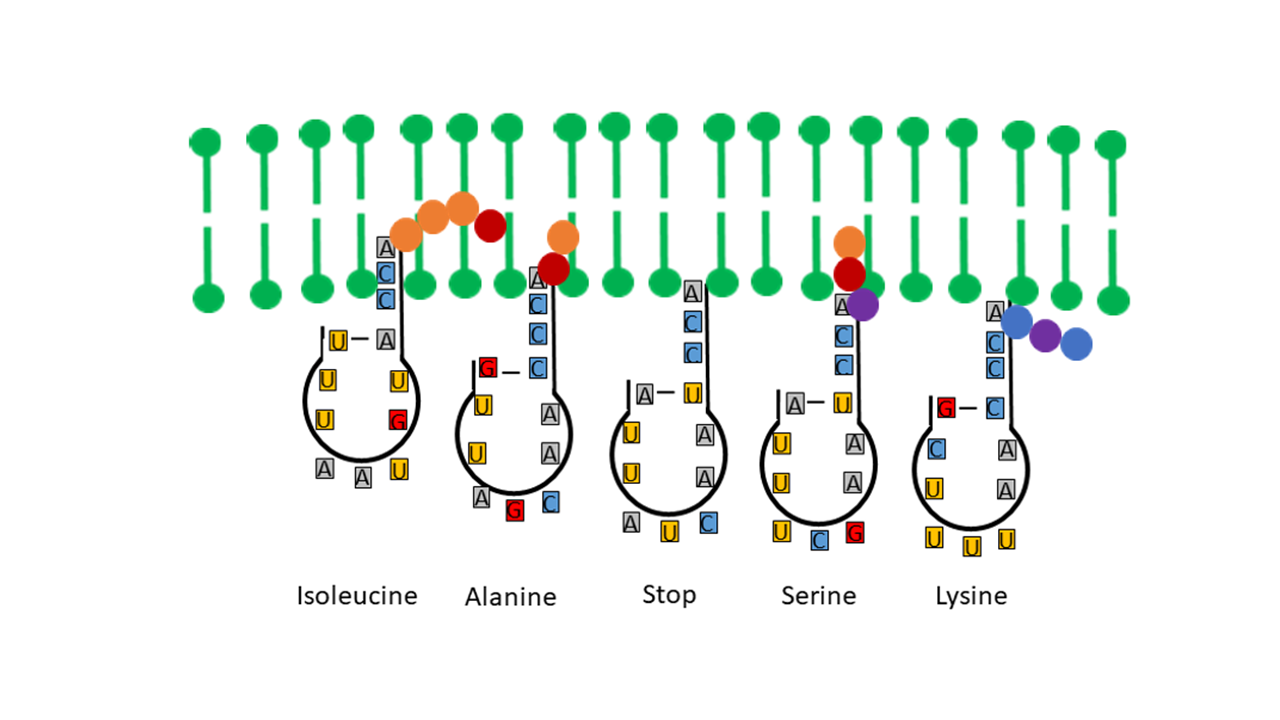
Fig. 11 Variation in Penetration Depths of the Acceptor Arm into the Membrane Depending on the Hydrophobicity of the Anticodon
This work is licensed under a Creative Commons Attribution-NonCommercial-ShareAlike 4.0 International License.
The question arises as to how a specific connection between the corresponding amino acid and the base triplet of the anticodon could have been achieved. At the base triplet, which ultimately dictates the code, three out of four possible bases are always present, differing in their hydrophobicity [10]. Depending on the types of bases involved and their positions within the triplet, different values for the entropic force emerge, contributing to varying depths of penetration of the acceptor arm into the membrane as a result of the hydrophobic effect (https://en.wikipedia.org/wiki/Hydrophobicity).
This means that the stronger the hydrophobicity in the region of the anticodon, the further the tip of the proto-tRNA protrudes into the membrane. There, it can be linked to a hydrophobic amino acid. The placement of short hydrophilic peptides in the membrane during the vesicle formation process is presumably more favorable than that of individual hydrophobic amino acids. The terminal amino acid of the peptides can be bound to the tip of the proto-tRNA while being simultaneously cleaved from the chain. Separating the amino acid from the chain increases entropy and thus favors the reaction. The linkage binds the amino acid at the 2'-OH position. With appropriate combinations of bases at the anticodon, different, finely tuned positions of the CCA arm in the membrane are achieved, thereby linking amino acids with varying hydrophobicities to different positions in the membrane (during a pressure drop with phase change of the gas). The probability of residence for the most hydrophobic amino acids is highest in the innermost zone. If more hydrophilic bases are present at the anticodon, the CCA arm does not extend as far into the membrane, leading to the linkage of hydrophilic amino acids at the 3'-OH position. No amino acids can be attached in a middle position. The corresponding combination corresponds to the current stop positions during mRNA decoding in the ribosome.
With these relationships, a process can be derived that allows for the storage of an amino acid sequence in an RNA by tightly occupying various proto-tRNAs on the inner surface of a vesicle membrane. The curvature of the membrane favors closely adjacent positions of the anticodons, allowing for complementary pairing of codons to form an RNA (proto-mRNA). However, a problem arises here regarding the chirality of the amino acids or ribose, for which a selection for a homochiral peptide is lacking. For longer chains, there are countless possible combinations, requiring corresponding periods of trial and error. A more effective process is achieved when alternatively the proto-tRNA is cleaved at the single double bond. It then exists as a single strand and can assume the function of a proto-mRNA (see below).
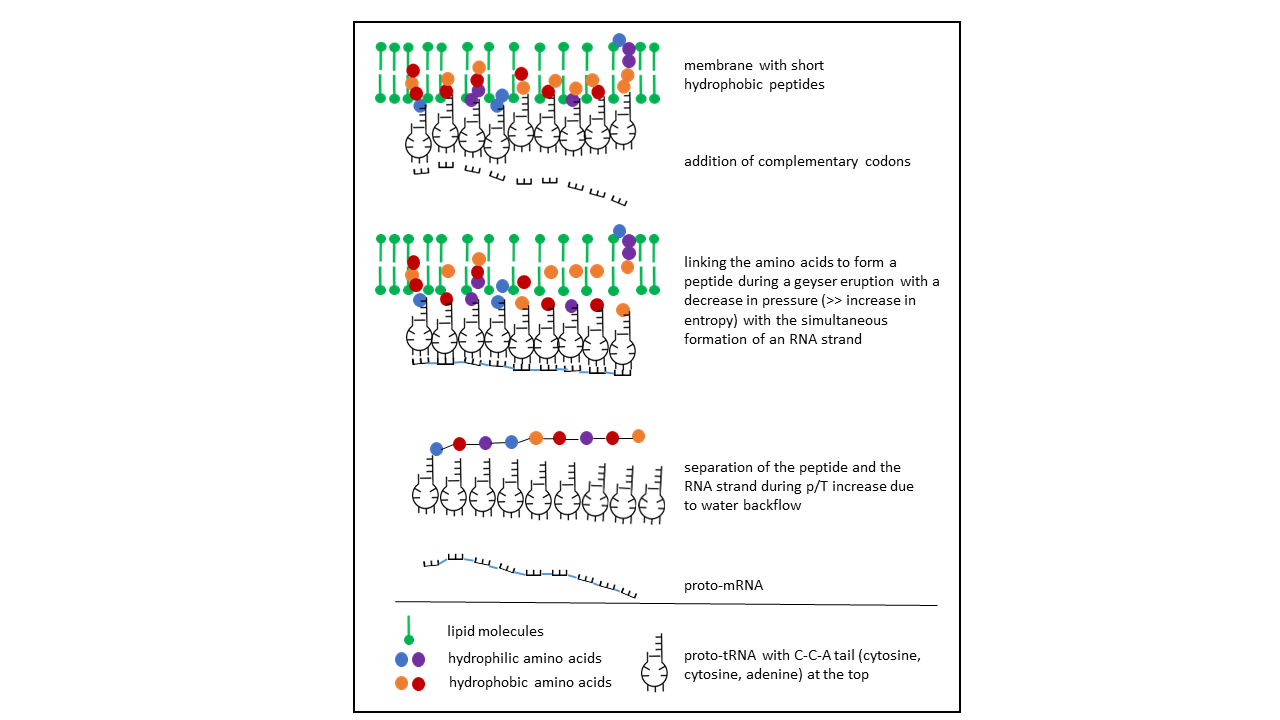
Figure 12 Submergence of Proto-tRNA into the vesicle membrane with the CCA acceptor arm at the top, depending on the hydrophobicity dictated by the anticodon. After the amino acids (hydrophobic AA yellow and red are bound to short peptides in the membrane) are linked to the proto-tRNA, the amino acids separate from the short peptides and link together to form a longer peptide.. The closely spaced anticodons serve as the information storage for the peptide sequence and act as a template for a Proto-mRNA, which forms by attaching complementary nucleotides and then detaching.
This work is licensed under a
Creative Commons Attribution-NonCommercial-ShareAlike 4.0 International License.
If the terminal ribose is at the level of the lipid heads on the inner edge of the membrane, there is no loading with an amino acid. The positions here correspond to the stop codons. In Table 1, when comparing the anti-codon assignments with the associated amino acids, it becomes clear that all hydrothermally formable hydrophobic amino acids have an adenine in the middle position or others in the edge area. The hydrophilic amino acids have a correspondingly opposite occupancy. Weber and Lacey [17] already discovered this striking correlation in 1978, without being able to offer an explanation for it.
Table 1 Left Side according to Jungck, J. R. (1978)[22]: This table provides a clear illustration of the relationships. Depicted are the relationships of three out of the four bases of each anti-codon to the encoded amino acid, depending on hydrophilicity (the first base on the left is the Wobble position). Amino acids that can be formed in hydrothermal systems are highlighted in color (exception: red for stop positions). Hydrophilic amino acids (shades of blue) are located in the corner with the most hydrophilic anti-codons. In contrast, Table 1 (right side) is presented for comparison. A sufficient explanation for these clear correlations has not been provided to date.
Table 1 Right Side: Canonical amino acids with decreasing hydrophobicity according to Kyte and Doolittle [20]. Hydrothermally formable amino acids are color-highlighted. For hydrophobic (hydrothermal) amino acids, the second position of the anti-codon on the tRNA (lower end) is occupied by the hydrophobic adenine (in red). In contrast, the tRNAs of hydrophilic amino acids possess the hydrophilic bases G, U, or C in this position.
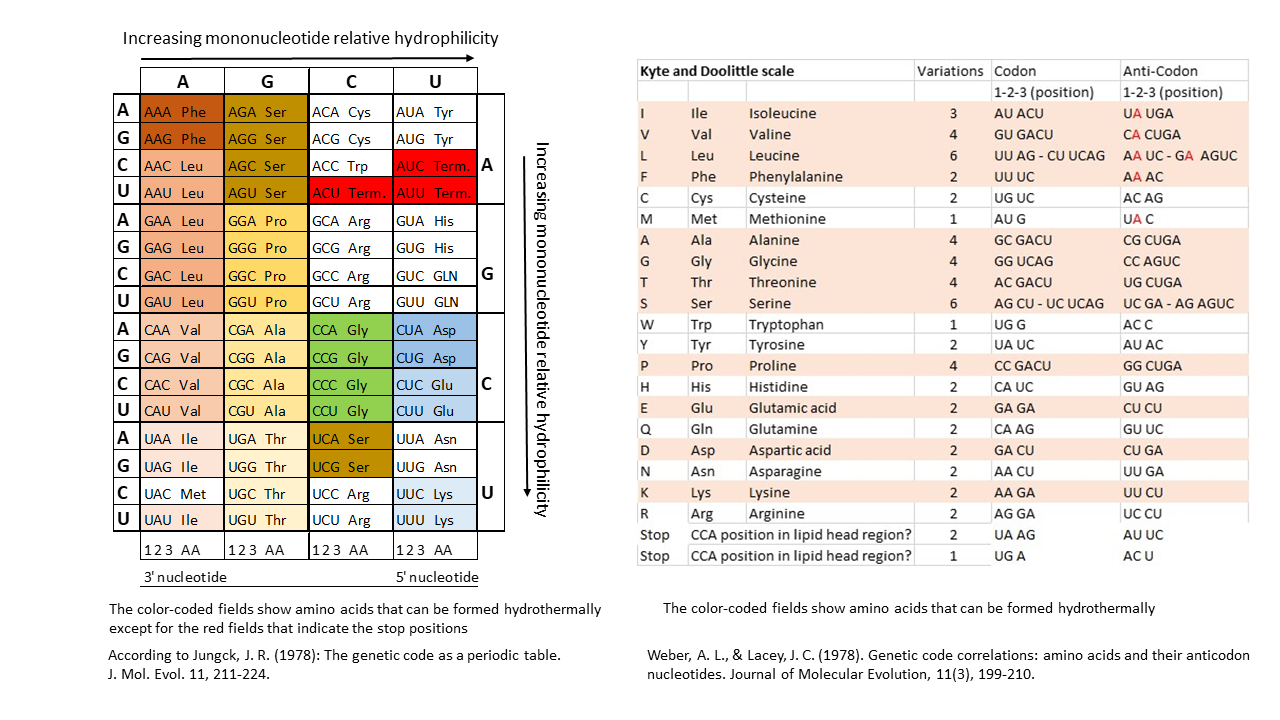
The different proto-tRNAs could be relatively specifically linked with amino acids based on the hydrophobicity of the anticodon. At the same time, the sole double bond of the tRNAs could be easily cleaved, resulting in a single strand. This single strand represented an informational strand that initially had no significance due to its random nucleotide sequence. Since loaded tRNAs were present at the same time, they could utilize the single strand as a proto-mRNA and form a tetrapeptide, provided suitable conditions existed. Some of the "tRNA templates" could not be used for a peptide because they provided sites for amino acids that did not yet exist. This also applies to the complementary strands that arise during replication (which is considered a prerequisite for the survival of the proto-tRNAs) (Fig. 13). After replication, the CCA segment, for example, represents the amino acid tryptophan in the complementary strand, which likely was not formed hydrothermally and only later took over the UGG code. By analogy, UGG might have originally represented threonine.
Since the proto-tRNA must have existed in a homochiral form (both as D and L versions) to be formed as such, and there is a preferential linkage of L-amino acids with D-RNAs (and vice versa) [23], the formed peptides were also homochiral (with both handednesses present in equal amounts). Since a maximum of 12 different amino acids could have existed with different proto-tRNAs, of which there could be several for one amino acid, a variety of different templates for tetrapeptides were available.
The linking of two tetrapeptides with the same handedness resulted in lengths of membrane thickness. This could result in the formation of pores that are necessary for exchange of ions and molecules or can already be used to generate energy. Since the sequences of the peptides were already stored, it only depended on the combination of the tetrapeptide chains or the proto-mRNAs to obtain the first functional molecules that were catalytically active. If these contributed to faster formation of further functional and other "useful" molecules, they won the race for resources and established their handedness.
If this process involves a molecule for the first time that possesses catalytic function and that remains as the core of more complex peptides in the further course of development, this can be considered as the beginning of life.
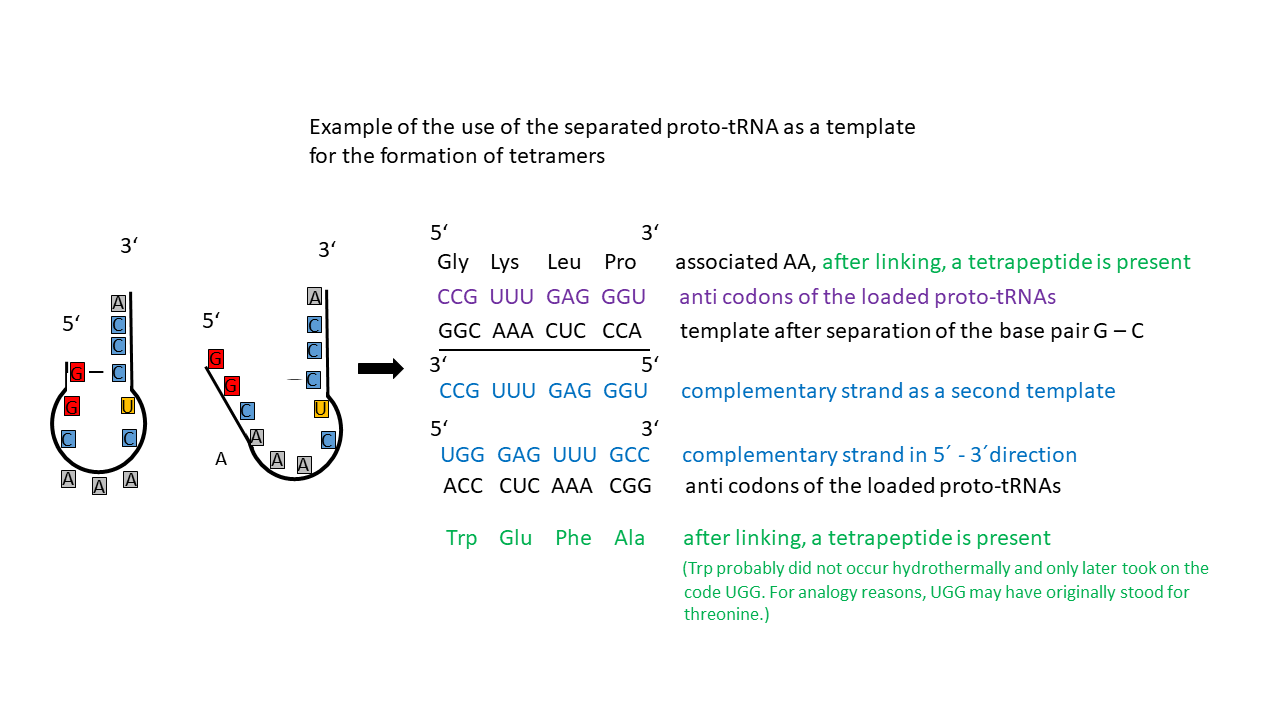
Fig. 13 Proto-tRNA as a single strand that can act as a template. Another template is created from the complementary strand. Tetramers can be formed from both, which are homochiral due to the tRNA.
This work is licensed under a Creative Commons Attribution-NonCommercial-ShareAlike 4.0 International License.
Advantage of using the opened proto-tRNA as a template:
If both the single strands of the tRNA (provided that the codes correspond to the hydrothermal amino acids) and their complementary strands are used as templates, catalytically active peptides can be generated from them. These peptides could later be encoded in DNA with bidirectional genetic coding (sense/antisense orientation).
As mentioned above, there are two classes of synthetases responsible for the specific loading of tRNAs. Amino acids associated with Class I synthetases, which are linked to the 2'-OH end of the terminal ribose, are generally larger and less polar, while those associated with Class II synthetases, which are attached to the 3'-OH end, are smaller and more polar. Biochemical, bioinformatic, and protein engineering experiments support the hypothesis that these two classes originate from opposite strands of the same ancestral gene [25]. This would support the idea that the basis for the evolution of today's aminoacyl-tRNA synthetases dates back to a very early phase, which is related to the template formation described above.
Table 2: Complementary groups of amino acids that can exhibit a relationship in the use of templates from proto-tRNAs and their complementary strands.
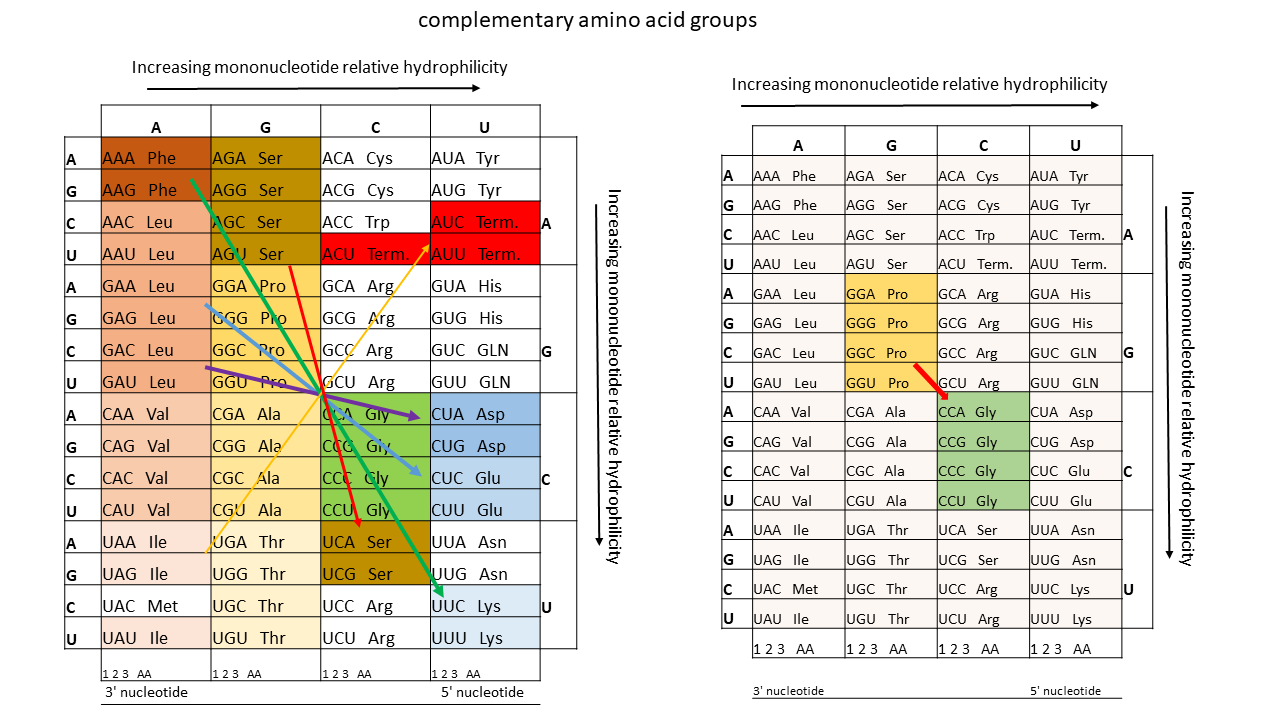
From a mechanical perspective, fixing a finger in a plastic medium is more favorable when the tip is thickened (similar to inserting a lollipop into a firm pudding). With the base combination "ACC" at the acceptor arm, adenine, which has two ring structures (purine scaffold), is followed by cytosine, which consists of two smaller bases with only one ring structure each (pyrimidine scaffold). This combination has likely proven to be the most advantageous (Fig. 14).
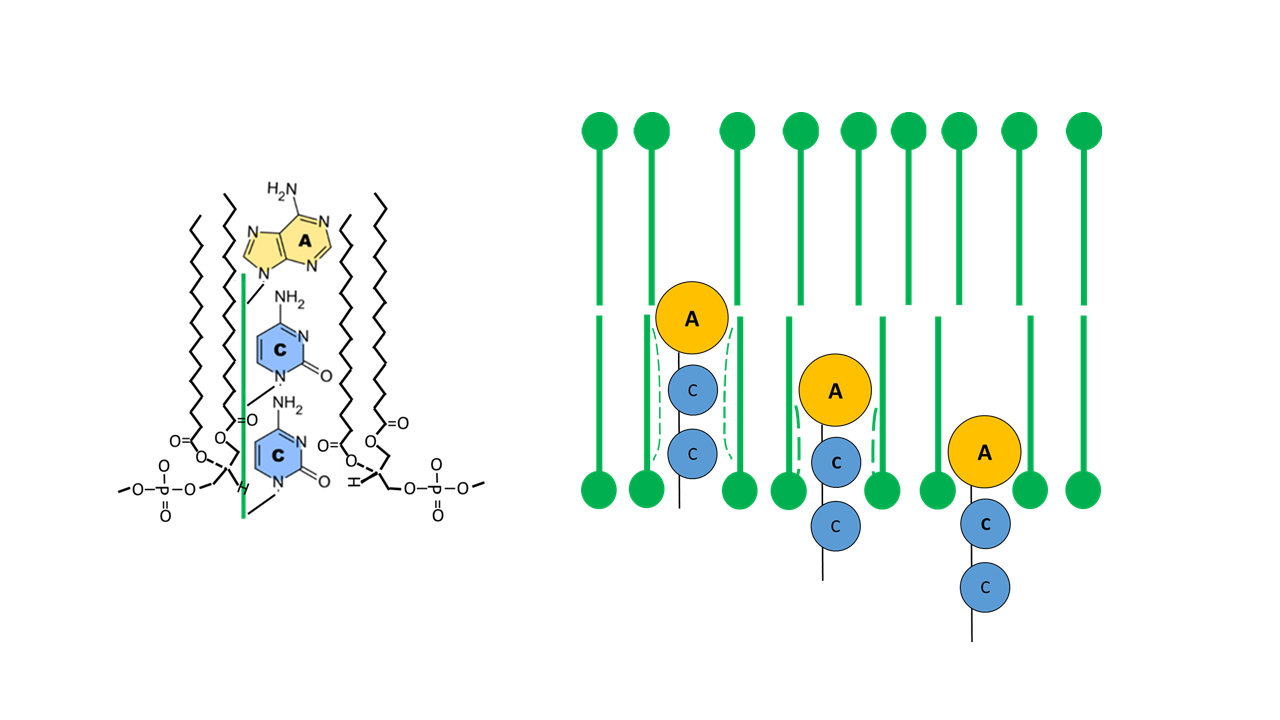
Fig. 14 Fixation of the ACC arm in the membrane (Lollipop structure for optimal integration, function of the membrane as a catalyst)
This work is licensed under a Creative Commons Attribution-NonCommercial-ShareAlike 4.0 International License.
During the chemical evolution leading to the "ACC" arm, numerous variations must have emerged that were unfavorable due to molecule size and/or degree of hydrophobicity (Fig. 15):
With guanine at the tip, there is a hydrophilic base that blocks immersion into the membrane, regardless of the nucleic bases that follow. With adenine at the tip and the smaller pyrimidine base uracil, the penetration of the acceptor arm into the membrane is possible. However, uracil is more hydrophilic than cytosine, making the combination with cytosine more favorable. If adenine is present not only at the tip but also at other positions, the fixation in the membrane becomes too strong, preventing fine-tuning of the penetration depth by the anticodon.
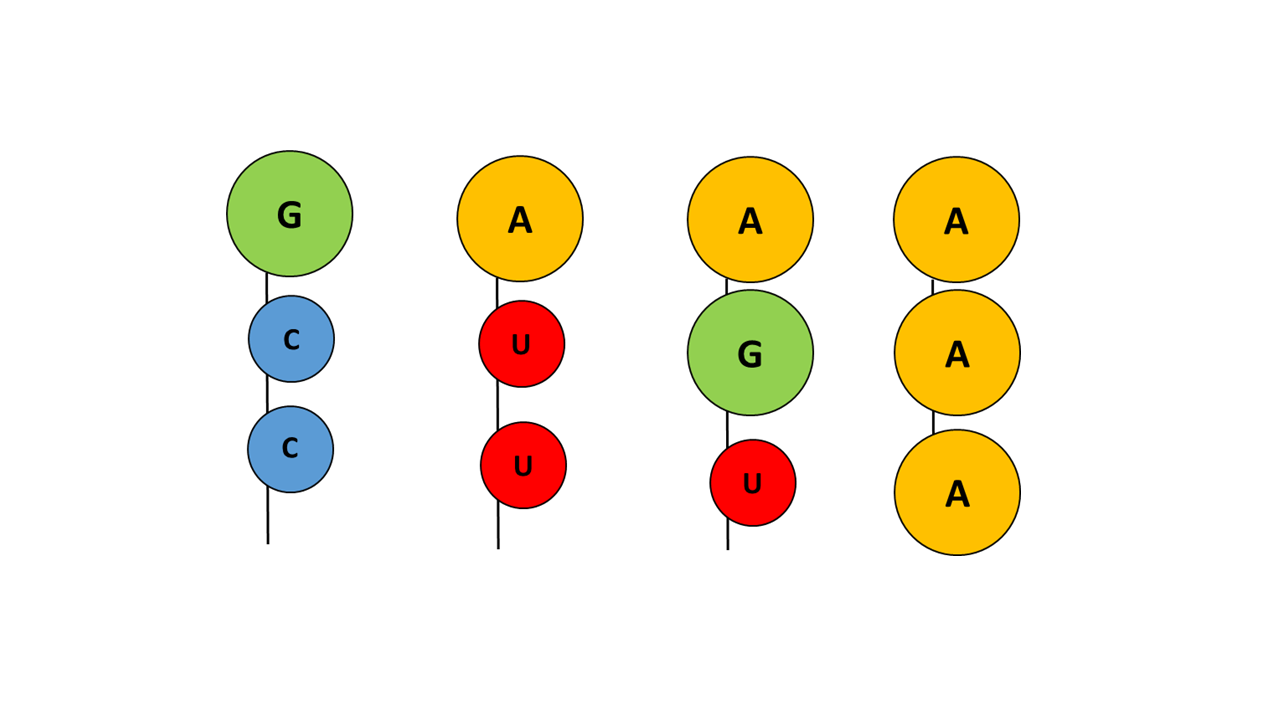
Fig. 15 Variations of different base combinations of the acceptor arm that have not prevailed
This work is licensed under a Creative Commons Attribution-NonCommercial-ShareAlike 4.0 International License.
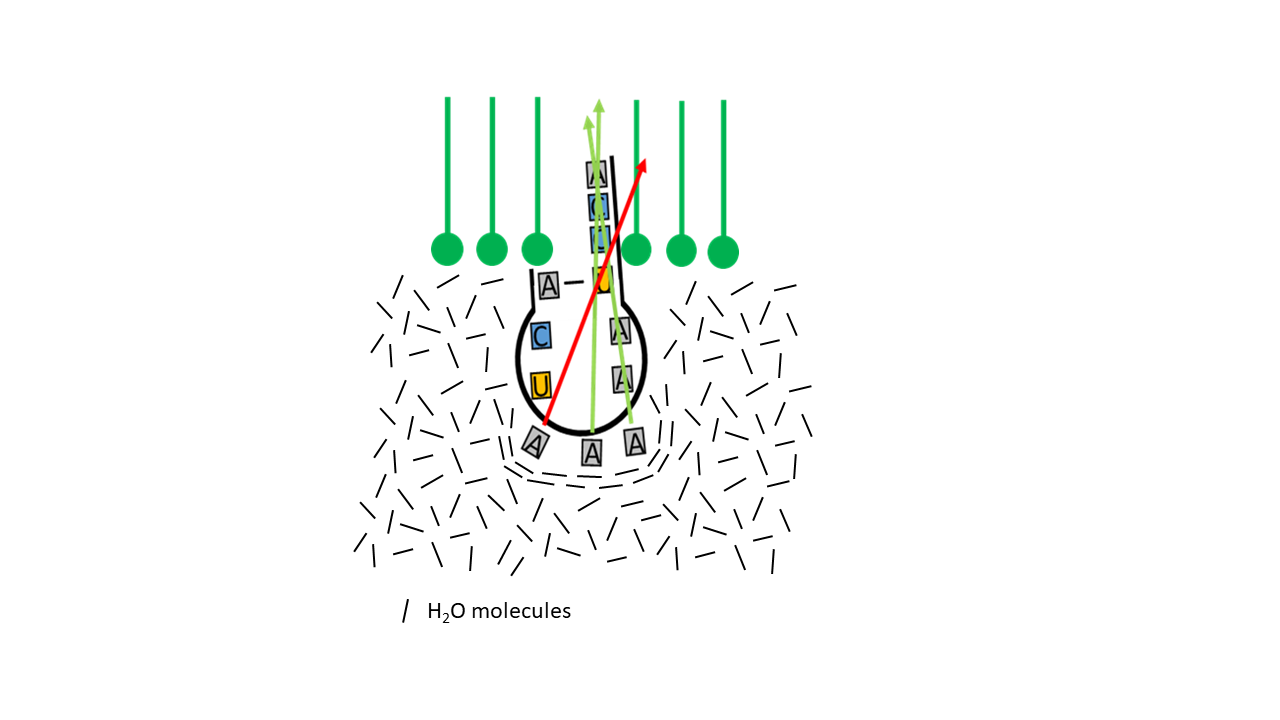
Fig. 16 Direction of the force vectors of the hydrophobic/entropic effect depending on the molecular structure
Specificity of the Genetic Code
One notable feature regarding the specificity of the genetic code is evident in the variability of the third base of the codon in mRNA (5' -> 3') and that of the anticodon (3' -> 5'). For triplets coding for the same amino acid, there is often only a difference in the third base (→ Wobble pairing). Consequently, the significance of the third base for specificity is less than that of the first and second bases. It is worth considering whether there might be a correlation with the hydrophobic/entropic effect.
For example, when considering the forces acting on a membrane-associated proto-tRNA using force vectors (Fig. 16), the molecular configuration results in the influence of the first two bases on the penetration direction of the acceptor arm being greater than that of the third base.
For suggestions, corrections, or discussions: cogita.ama@gmail.com
Hydrophobicity of tRNA
Suga et al. (2012) determined the hydrophobicities of modern tRNAs in an aqueous two-phase system at different temperatures [24]. It was found that below 40°C, the molecules were generally hydrophilic, while above 50°C, they were hydrophobic. Additionally, due to the higher temperatures, base pairs were disrupted, and the tRNAs denatured.
In the context of geyser eruptions, as described above, a cyclic temperature change within the cavities at the gas phase transition zone is expected. This temperature range may correspond to that used in the experiments by Suga et al. This allows for the discussion of certain consequences for the development of proto-tRNA:
At higher temperatures: single-strand formation, aggregation through hydrophobic interactions, facilitation of the copying process.
At lower temperatures: base pair formation, penetration of the acceptor arm into the membrane.
For suggestions, corrections, or discussions: cogita.ama@gmail.com
The Emergence Toward Prokaryotes
The combination of various individual strands of tRNAs, each consisting of 12 nucleotides (Nt), resulted in different evolutionary developments. Proto-tRNAs could be extended, thereby improving the specific linkage of amino acids. Concurrently, longer templates of these tRNAs enabled the formation of longer peptides, leading to the emergence of more complex enzymes. Among these were the aminoacyl-tRNA synthetases.
Other combinations of these single strands led to the formation of units that eventually, together with peptides, contributed to the development of the ribosome (5S rRNA (120 Nt): 10 strands; 23S rRNA (approximately 2900 Nt): about 240 strands; 16S rRNA (1500 Nt): 125 strands).
From a certain point in time, biochemical selection resulted in the cells being equipped in such a way that, with the supply of external components, all the necessary building blocks could be reproduced. After the proto-cells had grown sufficiently, physical forces (shearing during flow turbulence) were sufficient to cause the cells to divide. The daughter cells could continue to develop if there was a surplus of building blocks in the mother cell beforehand, which were still present in sufficient numbers in both daughter cells after division.
Already here there was a large number of starter cells, all of which had different (quantitative) equipment, but possessed the same "language and grammar".
Overview of the development of the first cells
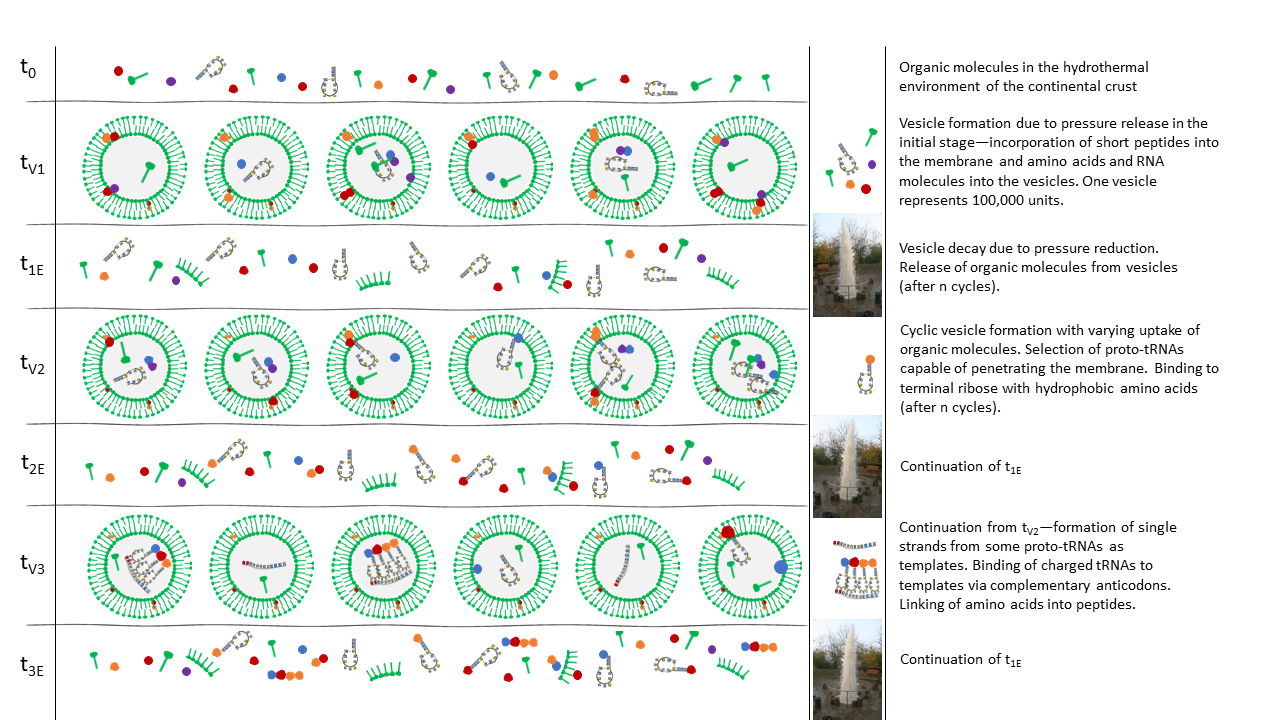
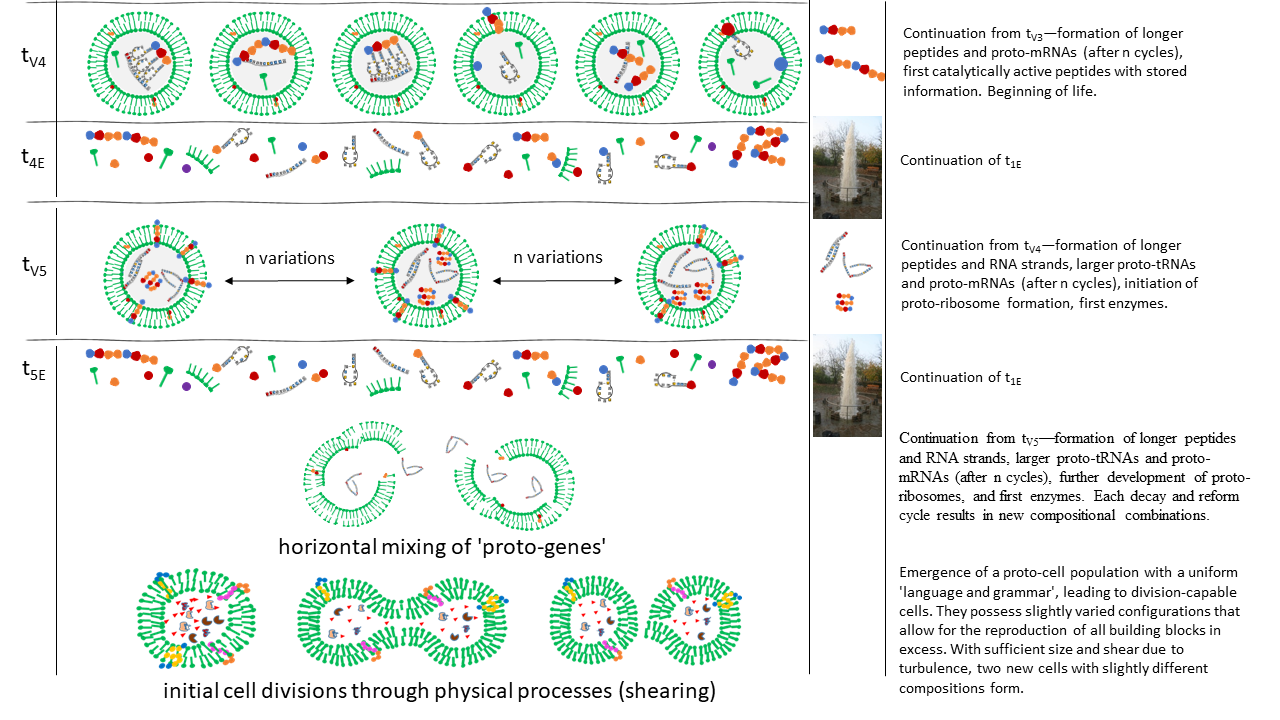
Fig. 17 depicts relevant time periods for the development of the first cells. Colored dots represent different amino acids. The green half-dumbbells are lipids forming the vesicle membrane, and the curved RNA strand symbolizes various proto-tRNA variations, including a specific single-strand variant. The bars labeled 't' indicate developmental times ranging from thousands to millions of years, with tV phases (vesikel) alternating cyclically with tE phases (eruption). A vesicle in a bar represents approximately 100,000 vesicles formed during a pressure drop during a geyser eruption. As development progresses, the first catalytically active functional molecules appear, with information stored in RNA. At this point, the chirality of the subsequent molecules is determined. It can be defined as the beginning of life. (tV4). Each cycle reassembles the building blocks of life in proto-cells. Once the first cells reach a level of organization that allows for self-sustaining building block formation with adequate external supply, cell division can commence.
This work is licensed under a Creative Commons Attribution-NonCommercial-ShareAlike 4.0 International License.
What happened next?
The geyser eruptions continuously carried organic molecules and eventually the first self-replicating cells to the surface. Consequently, "bio-films" made of organic materials must have formed in the vicinity of the exit points, in which the first cells were also embedded. However, they had little chance of surviving under the new environmental conditions. UV exposure, solar wind, lower temperatures and higher pH levels required an adaptation that took a longer period of time.
In addition, the energy and substance supply was difficult. However, the biofilm in the vicinity of the geyser probably provided the conditions for the cells to be able to absorb substances from outside. Sooner or later, climatic or regional conditions may have made it possible for the cells to survive on the earth's surface, so that they could reach the oceans via rivers. An alternative situation is conceivable for locations close to the coast, whose open fault systems lead directly into the marine environment. Entering the ocean was the decisive step that set the system of life in motion. In the ocean, the cells encountered areas that were familiar to them in terms of physicochemical conditions. It was the Black Smokers and other hydrothermal vents that provided them with unlimited, usable energy resources. At the same time, this environment again offered protection from the destructive influences that were present on the surface of the earth.
The appropriation of the submarine hydrothermal vents by the first prokaryotic cells probably led to an explosive spread of the first life form, viewed in a compressed time period. The open ocean offered ideal conditions for this with sea currents and a sufficient number of hot springs.
Long distances and unique local characteristics could lead to independent adaptations in the young family of cells, which quickly diversified as a result. This phase marked the end of the violent meteorite impacts, which mainly affected the oceans. Every major impact caused large areas of the young continents to be flooded. This was the way in which the prokaryotes were able to spread over all the large and small fragments of continental crust that had now been formed. Wherever they came into contact with hydrothermal vents on land, they could gain a foothold and continue developing.
In this context, another development on the young Earth is significant. The movement of plates began early on, which ultimately led to the shifting of the continents and subsequent collisions. What influence did this process have on the further development of the prokaryote?
The contact between differently developed cells
It is now accepted that organelles such as the mitochondria and chloroplasts of eukaryotes are derived from bacteria. There is an idea that bacteria were taken up by other prokaryotes but could not be digested. On the contrary, the absorbed bacteria continued to live in the host's cell envelope and used their metabolic products for their own sustenance. An endosymbiosis developed in which one partner lives permanently in the body of the other, like the bacteria in the intestine. The evolutionary adaptation meant that the "undigested" bacteria were gradually reduced to useful building blocks that today make a decisive contribution to the energy supply of the cell.
The eukaryotes appeared almost spontaneously on the stage of life 1.5 billion years ago. Ancestors that could be considered as transitional stages have not yet been clearly identified. 500 million years earlier, almost all existing small continents began to migrate towards one another, until a supercontinent had formed 300 million years later. It is referred to by geologists as Columbia. Its existence lasted for another 200 - 300 million years, whereby the last 100 million years were already characterized by decay and mountain building processes caused by plate tectonics. Finally, in the final phase of Columbia, the eukaryotes appeared. A billion years earlier, the concentration of oxygen in the atmosphere began to increase, to which the living world had to react.
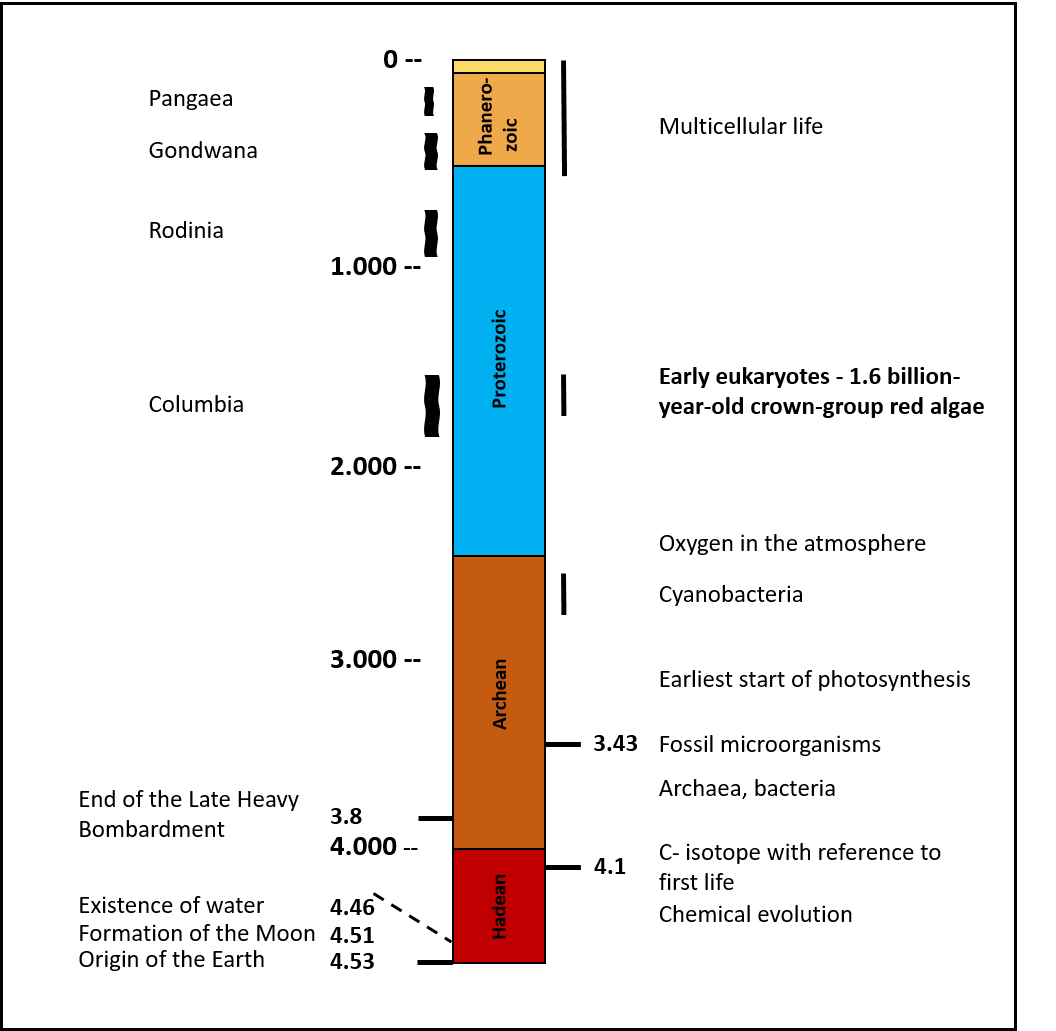
Fig. 12 Time scale of earth evolution - Age of eukaryotes after Bengtson et al. 2017 [21]
It can be assumed that endemic bacterial strains developed on all small continents far apart over more than 1 billion years, which from a certain point in time over millions of years gradually converged on one large continent. Each time a new small continent landed at the large complex, different specialized bacterial species "suddenly" confronted each other. The number of individuals and time period were large enough for all variations of cohabitation to be tried out. It cannot be excluded that this process led to the development of the endosymbionts, perhaps even several times as a result of small continents subsequently arriving, as can be observed today in some cell organelles (plastids of brown algae, gold algae and diatoms). If the adaptation to the increasing oxygen concentrations in the atmosphere and the ocean water ran parallel to this, everything taken together could explain the start of the eukaryotes at the end of Columbia.
This hypothetical scenario immediately raises a number of questions. Are there still relics in the DNA of bacteria and archaea that point to the time of endemic development? Can provinces be found on the continents that correspond to ancient continent cores and are dominated by such bacteria, perhaps in the deep biosphere?
Findings:
(Hypothesis on the Origin of Life in Bullet Points)
Life originated according to the model in the young continental crust at a depth of less than 1000 meters, where the transition from supercritical to subcritical gases occurred and continues to occur today.
From the ascending gases and decomposed minerals, organic molecules could be formed under variable pressure and temperature conditions of the crust, with unlimited resources.
Clay minerals from altered crustal rocks and/or colloidal SiO2, as well as other mineral surfaces, likely catalyzed the formation of short RNA strands, among which a specific type, the Proto-tRNA, was selected as the key molecule.
The Key Molecule: The Proto-tRNA
A Proto-tRNA constructed with a minimal number of nucleotides (12) is considered the key molecule for information storage. It features a single strand with the CCA base sequence on the acceptor arm, capable of binding a specific amino acid, and a loop on the other side containing the anticodon, consisting of three outward-facing bases. With adenine (A) at the tip of the acceptor arm, a hydrophobic base is present, enabling the arm to penetrate a membrane. Different hydrophobicities are achieved depending on the arrangement of nucleobases in the anticodon triplet.
A selection factor was the stability of the chains. Hydrolysis countered the formation of long chains. An optimum length of 12 nucleotides might have been proven if loop binding increased stability by forming only one additional base pair.
A flotation-like transport system, induced by the ascending supercritical gases, shifted molecules to the upper crust, where they accumulated in high concentrations in the phase transition zone at a depth of approximately 1000 meters.
Cyclical pressure fluctuations, controlled by outbreaks of cold-water geysers (overlaid by strong earth tides), led to the formation of vesicles in the transition zone, filled with supercritical gases and water. These vesicles enclosed hydrophilic molecules inside and hydrophobic ones in the membrane.
With pressure fluctuations came cyclical temperature variations, enabling the melting and copying of double-stranded RNAs.
The initial information storage occurred in the vesicles in conjunction with the unique properties of the membranes.
Integral to the first information storage were the varying hydrophobicities of amino acids and nucleobases.
The selected Proto-tRNA established connections to different amino acids via the depth of penetration of the acceptor arm into the membrane, each located at the beginning of short peptides. Hydrophobic peptides resided in the membrane, hydrophilic ones in the vesicle water. The depth of penetration was influenced by the differing hydrophobicity of the anticodon depending on the nucleobase occupancy.
The controlling factor was the entropic/hydrophobic effect.
One method of information storage involved creating templates through closely spaced Proto-tRNAs penetrating the membrane. The curvature of the vesicle membrane provided the conditions for configuring such templates, where complementary nucleotides could attach, forming a proto-mRNA. Like the proto-tRNA, the proto-mRNA could subsequently be copied. However, it should be noted that the existence of both handednesses in both RNA and amino acids would have led to many "unusable" mixed variants.
An effective alternative could be the use of P (primary) proto-tRNA or its complementary S (secondary) strand as a template for peptide formation. The only base pairing between the loop and acceptor arm could be easily separated, transforming the proto-tRNA into a single strand, now usable as P proto-mRNA. Possibly, the number of different proto-tRNAs matched the number of hydrothermally formable amino acid species (12).
Through replication of the P proto-mRNA, the complementary S strand gave rise to the actual template for the formation of tetrapeptides, which could be recognized by the loaded original proto-tRNAs.
Peptides formed from the single strands of comlementary S proto-mRNA were homochiral, with both handednesses occurring equally frequently.
With the use of proto-mRNAs, the sequence of the four linked amino acids (tetramers) was already determined. It was random and depended on the nucleotide sequence of the respective proto-tRNA. Combinations of different tetramers (each with the same handedness) from different templates could form catalytically effective peptides or membrane peptides, which beneficially influenced further development.
With the formation of the first catalytically effective molecule, whose sequence was stored in RNA, the start of life was accomplished. A prerequisite for defining this moment as the starting point is that the information about the peptide sequence was retained, allowing the molecule to continue being formed and contributing to the catalysis of further functional molecules.
There was no isolated RNA-first, peptide-first, or metabolism-first world (In a figurative sense, one could describe them as dead ends through inbreeding). Instead, three key players must have coexisted, undergoing chemical evolution in mutual interdependence: vesicles with their membranes, peptides, and the smallest possible proto-tRNA. The membrane acted as a catalyst, with differences in the hydrophobicity of amino acids and nucleobases playing a significant role in information storage, in conjunction with entropic effects. The single strands of tRNA served as the information storage medium, which only later gained significance following the selection of functional associated peptides.
Pressure fluctuations in the disruption zone caused cyclic releases of molecules into the cavities through vesicle decay, with the possibility of further development and incorporation into newly forming vesicles. There was a constant exchange between vesicles and the open system.
From the moment when cell membranes allowed exchange with the environment through pores and a variety of catalytically effective building blocks were present in the proto-cell, allowing growth and replication of the building blocks, cell division through physical processes (shearing by turbulent geyser outbreaks) became possible. This was the breakthrough for the proliferation of the first cells.
The processes in the crust continued parallelly even after the formation of the first self-replicating cell, meaning there were ongoing developments based on the same processes that could lead to self-replicating cells.
Thus, the development of life occurred in a continuous process theoretically ongoing to this day, constantly interlinked with evolving life, at least in the early phase. From this perspective, the question of why life did not originate multiple times does not arise.
The emergence of life through similar processes on other planets is highly probable, probably with not very different organic chemistry if the chemical composition of the planets is comparable.
Life did not go against the natural increase of entropy. The increase in entropy was the controlling factor in development.
And Chirality?
The evolution of chemical evolution to D-ribose in RNA and L-amino acids in peptides could possibly be explained by a random excess of one enantiomer at a specific time, which is, however, difficult to comprehend. Essentially, equal amounts of molecules exist in the D- and L-versions, so all reaction steps in the initial phase must have occurred with both. However, this is only until the point when the first catalytically effective peptide emerged, stored in RNA, and significantly influenced further development (start of life).
It ensured that resources were more heavily consumed in this group (today's D-ribose and L-amino acids) due to the faster formation of successor molecules. It is almost impossible that such a peptide emerged in both the D- and L-versions at exactly the same time.
literature
[1] Schreiber, U., Locker-Grütjen, O., & Mayer, C. (2012). Hypothesis: Origin of life in deep-reaching tectonic faults. Origins of Life and Evolution of Biospheres, 42(1), 47-54.
[2] Mayer, C., Schreiber, U., & Dávila, M. J. (2015). Periodic vesicle formation in tectonic fault zones—an ideal scenario for molecular evolution. Origins of Life and Evolution of Biospheres, 45(1), 139-148.
[3] Dhuime, B., Hawkesworth, C. J., Cawood, P. A., & Storey, C. D. (2012). A change in the geodynamics of continental growth 3 billion years ago. Science, 335(6074), 1334-1336.
[4] Mayer, C., Schreiber, U., Dávila, MJ, Schmitz, OJ, Bronja, A., Meyer, M., Klein, J. & Meckelmann, SW (2018). Molecular evolution in a peptide-vesicle system. Life, 8(2), 16.
[5] Schreiber, U., Mayer, C., Schmitz, O. J., Rosendahl, P., Bronja, A., Greule, M., Keppler, F., Mulder, I., Sattler, T. & Schöler, H. F. (2017). Organic compounds in fluid inclusions of Archean quartz—Analogues of prebiotic chemistry on early Earth. PLoS One, 12(6), e0177570.
[6] Großmann, Y., Schreiber, U., Mayer, C., & Schmitz, O. J. (2022). Aliphatic Aldehydes in the Earth’s Crust—Remains of Prebiotic Chemistry?. Life, 12(7), 925.
[7] Benner, S. A., Kim, H. J., & Carrigan, M. A. (2012). Asphalt, water, and the prebiotic synthesis of ribose, ribonucleosides, and RNA. Accounts of chemical research, 45(12), 2025-2034.
[8] Pedreira-Segade, U., Feuillie, C., Pelletier, M., Michot, L. J., & Daniel, I. (2016). Adsorption of nucleotides onto ferromagnesian phyllosilicates: Significance for the origin of life. Geochimica et Cosmochimica Acta, 176, 81-95.
[9] Cleaves II, H. J., Scott, A. M., Hill, F. C., Leszczynski, J., Sahai, N., & Hazen, R. (2012). Mineral–organic interfacial processes: potential roles in the origins of life. Chemical Society Reviews, 41(16), 5502-5525.
[10] Shih, P., Pedersen, L. G., Gibbs, P. R., & Wolfenden, R. (1998). Hydrophobicities of the nucleic acid bases: distribution coefficients from water to cyclohexane. Journal of molecular biology, 280(3), 421-430.
[11] Jarvinen, P., Oivanen, M., & Lonnberg, H. (1991). Interconversion and phosphoester hydrolysis of 2', 5'-and 3', 5'-dinucleoside monophosphates: kinetics and mechanisms. The Journal of Organic Chemistry, 56(18), 5396-5401.
[12] Mayer, C., Schreiber, U., & Dávila, M. J. (2017). Selection of prebiotic molecules in amphiphilic environments. Life, 7(1), 3.
[13] Eriani, G., Delarue, M., Poch, O., Gangloff, J., & Moras, D. (1990). Partition of tRNA synthetases into two classes based on mutually exclusive sets of sequence motifs. Nature, 347(6289), 203-206.
[14] Baillet, J., Desvergnes, V., Hamoud, A., Latxague, L., & Barthélémy, P. (2018). Lipid and nucleic acid chemistries: combining the best of both worlds to construct advanced biomaterials. Advanced Materials, 30(11), 1705078.
[15] Marshall, W. L. (1994). Hydrothermal synthesis of amino acids. Geochimica et Cosmochimica Acta, 58(9), 2099-2106.
[16] Hennet, R. C., Holm, N. G., & Engel, M. H. (1992). Abiotic synthesis of amino acids under hydrothermal conditions and the origin of life: a perpetual phenomenon?. Naturwissenschaften, 79(8), 361-365.
[17] Weber, A. L., & Lacey, J. C. (1978). Genetic code correlations: amino acids and their anticodon nucleotides. Journal of Molecular Evolution, 11(3), 199-210.
[18] Schreiber, UC (2019). The secret of the first cell: on the trail of the origin of life. Springer-Verlag.
[19] Schreiber, U. C., & Mayer, C. (2020). The first cell. Springer International Publishing.
[20] Kyte, J., & Doolittle, R. F. (1982). A simple method for displaying the hydropathic character of a protein. J Mol Biol, 157(1):105-132.
[21] Bengtson, S., Sallstedt, T., Belivanova, V., & Whitehouse, M. (2017). Three-dimensional preservation of cellular and subcellular structures suggests 1.6 billion-year-old crown-group red algae. PLoS Biology, 15(3), e2000735.
[22] Jungck, J. R. (1978). The genetic code as a periodic table. Journal of Molecular Evolution, 11, 211-224.
[23] Tamura, K., Schimmel, P. (2004). Chiral-selective aminoacylation of an RNA minihelix. Science, 305, 1253.
[24] Suga, K., Tomita, H., Tanaka, S., & Umakoshi, H. (2012). Hydrophobic properties of tRNA with varied conformations evaluated by an aqueous two-phase system. International Journal of Biological Sciences, 8(8), 1188.
[25] Carter, C. W. (2017). Coding of Class I and II aminoacyl-tRNA synthetases. Protein Reviews: Volume 18, 103-148.
The discovery of water on extra-terrestrial planets is as valid an indication of the potential presence of beer as it is of higher life.