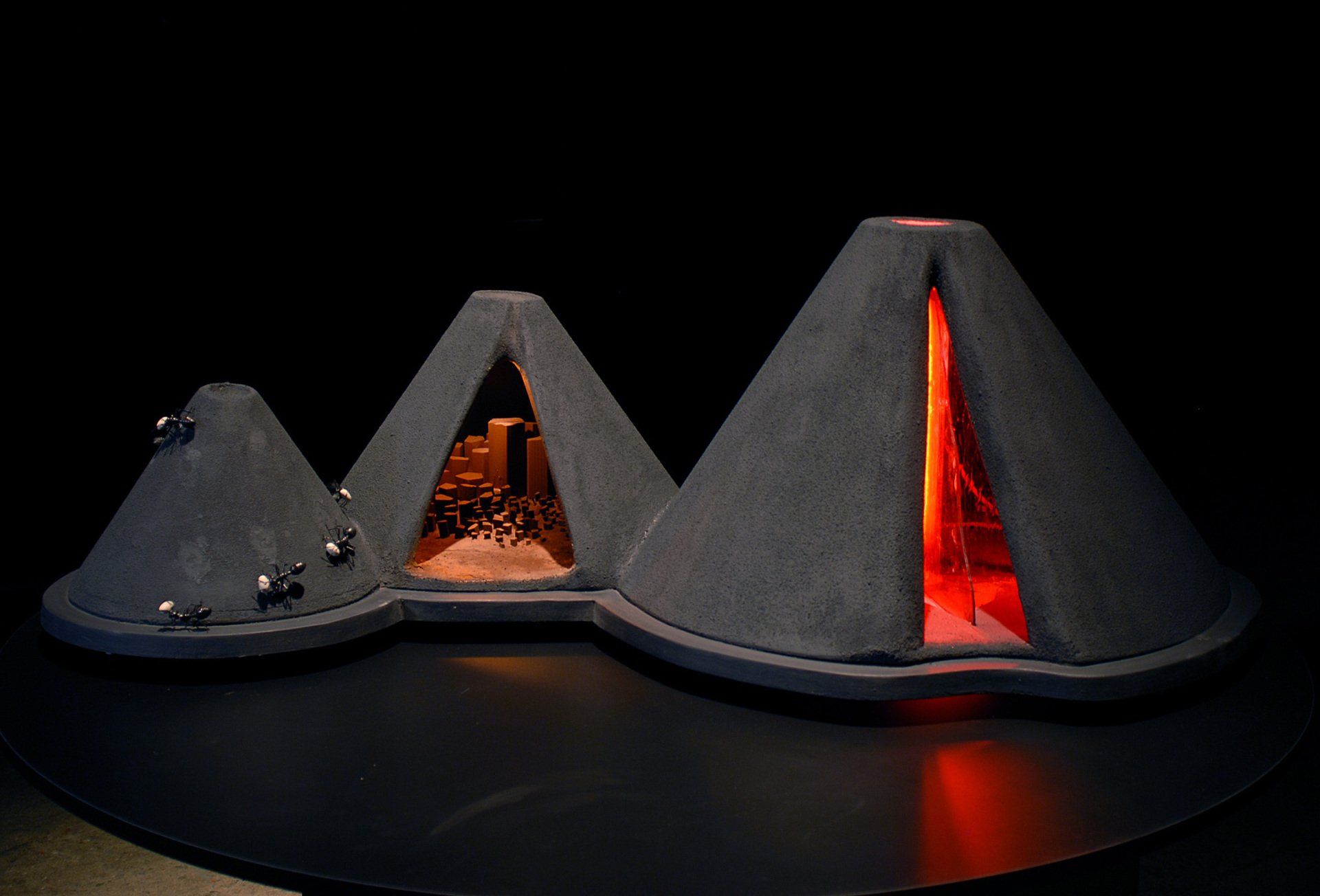
Volcanology
One of my research focuses was and is volcanism, which has been on my mind since my doctorate to this day. It started under the supervision of Prof. Klaus Schwab (Clausthal University of Technology) with the processing of volcanic rocks of the Puna in the Argentine high Andes near San Antonio de los Cobres (northwest of Salta, NW Argentina). This was followed by researching of the tertiary Westerwald volcanic rocks during my time as an assistant in geology at the University of Bonn, as well as investigations on the Mayon in the Philippines. When I was appointed to what was then the University of Essen, my research focus shifted to Eifel volcanism, with the reassessment of the Laacher-See magma chamber volume and all its consequences being the main focus. The paradox of a 0.5 cubic kilometer caldera with a postulated 6.5 cubic kilometers of ejected magma had to be questioned.
And then there is the problem of final storage of highly radioactive waste, which should be stored as safely as possible and without contact to volcanic activity at a safe depth. A study of the probability of a volcanic hazard in certain regions in Germany within the next 1 million years was carried out together with Prof. Gerhard Jentzsch on behalf of the Federal Agency for Disposal.
Volcanic hazard in Germany
Evaluation of possible volcanic activities in Germany over the next 1 million years, including determination of the areas with a probability of occurrence in this period.
Ulrich C. Schreiber1, Gerhard Jentzsch2
1Department of Geology, University of Duisburg-Essen, Essen, 45141, Germany, retd.
2Institute for Geosciences, University of Jena, Burgweg 11, 07749 Jena, Germany, retd.
Correspondence to: Ulrich C. Schreiber (ulrich.schreiber@uni-due.de) and Gerhard Jentzsch (gerhard.jentzsch@uni-jena.de)
Federal Society for Disposal (BGE), full report: https://www.bge.de/fileadmin/user_upload/Standortsuche/Forschung/Report_-_Vulcanic_Gefaehrdung_in_Deutschland_barrierefrei.pdf
Abstract
In connection with the search for a repository for radioactive, heat-generating waste in deep geological formations, future volcanic activity has to be taken into account when selecting a location in Germany. For an estimation of possible volcanic activity within the next 1 million years, geological/geochemical data, geophysical investigations and isotope data of crustal and mantle gases were evaluated. Teleseismic studies, monitoring results of seismic/microseismic activity through deep earthquakes, and geodetic investigations of vertical relative movements of the crust were also considered. Further criteria come from the fields of geology and mineralogy, which enable statements to be made about the distribution and origin of volcanic rocks. The age of volcanic deposits contains information about the process and magmatic developments within a volcanic field as well as possible volcanic activity cycles. Isotope ratios of gases from springs and mofettes provide clues to their origin and to the extent to which the mantle contributes to the composition of gases. Together with the geophysically determined mantle anomalies and the age of the volcanoes, they provide the basis for determining the areas for which there may be a probability of future volcanic activity.
Special attention was paid to the Quaternary volcanic regions of Eifel and Vogtland because there is a high probability of a resurgence in volcanic activity. The outer volcanoes of the resulting area provide reference points for an initial estimate of the region that has a probability of recurring activity. An extent with a radius of 15 km around a volcano is assumed at the surface, within which magma can escape when it rises again. In addition, a safety distance of 10 km is added, so that a distance of 25 km in total from the Quaternary eruption point to the outer border is ensured. In the Vogtland region, centres of earthquake swarms were set equal with the volcanoes and the size of the area for the probability of recurring volcanic activity was adjusted accordingly. A secondary effect of a volcanic eruption can result from the damming of the Rhine in its narrow valley by lava flows and tephras. Long-term damming of the Rhine would lead to extensive flooding in the upper Middle Rhine Valley, the Upper Rhine Graben and adjacent valleys. In addition to the Quaternary volcanic fields of Eifel and Vogtland, there are two regions in Germany for which there is a low probability of a volcanic eruption within the next 1 Myr. The first one lies north of the Westerwald and borders directly to the area with the high probability of occurrence of the Eifel. The second area lies between Stuttgart and the Lake Constance region. The Tertiary Urach volcanic field and the marginal area of the also Tertiary Hegau field are located there. For the remaining Tertiary volcanic fields in Germany, no volcanic activity can be assumed within the next 1 Myr due to their high age and lack of evidence from the mantle and gas compositions.
The recent development of the Eifel region (West and East Eifel Volcanic Field (WWVF and EEVF) and adjacent areas is determined by tectonic processes, fluid migration in the Earth’s crust and magmatic processes in the Earth’s mantle. This becomes obvious in the distribution of earthquakes epicenters and gas leakages of geogenic gases as well as in the concentrations of certain trace gases in mofettes and mineral springs. Research in the past focused on selected topics as, e.g. kinematic motion, uplift processes, stress field analyses or the volcanic evolution.
Latest accumulation of earthquakes and their distribution in the study area deserve special attention because of the recent volcanic evolution of the EEVF. This region will be the most likely area for a new volcanic activity.
For this reason, not only a comprehensive knowledge of the spatial and temporal characteristics of present-day tectonics, and earthquake events is important but also knowledge of the large previous volcanic eruptions is of urgent interest and very helpful in understanding the geodynamics of intraplate areas. The magma-tectonic relations, the size of the magma chamber and the amount of the erupted material provide first indications to identify new developments that may lead to new processes within the magma chamber. For that reason, the previous presentations of formation and dimension of the Laacher See magma chamber and the erupted volume have to be questioned and re-considered. Therefore, a comprehensive investigation was successfully conducted in the study area (cf. Figure 1), to investigate the correlation between active tectonic fault regimes, gas migration and the Laacher See magma chamber size and caldera formation in order to assess the seismic hazard of future volcanic activity in this area.
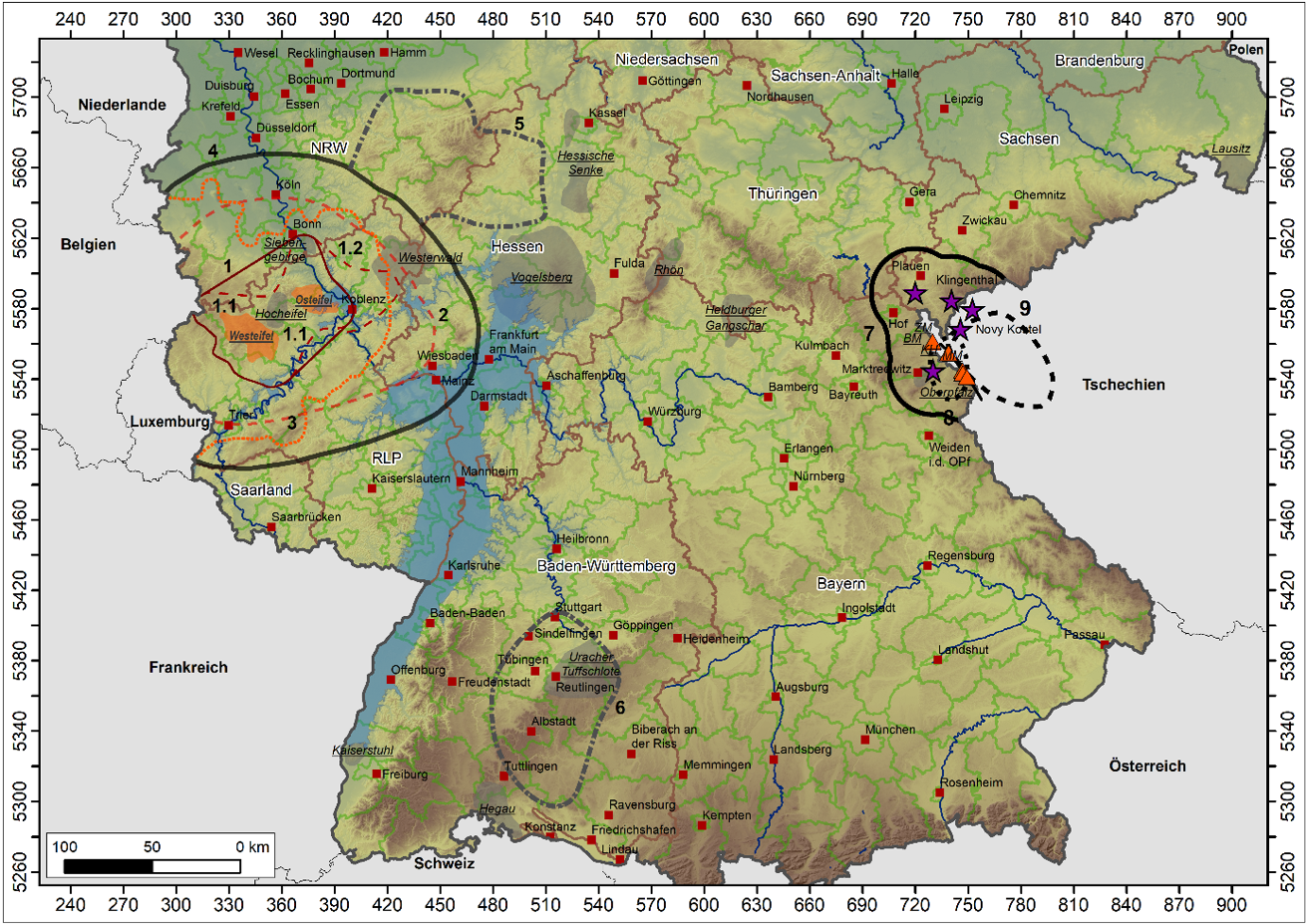
New discussion points on maar formation and the Laacher-See volcanism
(excerpt from: Volcanic hazard in Germany, Schreiber, Jentzsch, 2021)
9.1.1 Maars
The initial phase of the volcanic eruptions led in most cases to the formation of explosion funnels in the Eifel (Maar stage). In the case of an exclusive maar development, the activity presumably ceased after a few weeks to months. The maars were mainly formed in valleys in which contact with water through streams and aquifers was given during volcanic activity. Such contact causes phreatomagmatic eruptions which are generally seen as explanations for maar formation (Lorenz, 1973).
The ascent paths of magmas are tectonically controlled and are opened depending on the regional stress regime. Strike -slip faults provide the most favourable conditions for magma ascent. Depending on the state of stress or seismic activity, they are open and fluid-filled (e.g. supercritical water mixed with supercritical gases at greater depths) or closed by hydrothermally formed minerals. Bent trends of such faults lead to compression (transpression) or stretching (transtension). If displacement is rapid and sufficient, channels can develop through the entire crust up to the mantle, creating pathways for rapid ascent and transport of magma.
Depending on the volume of the rising magma and the stress situation in the crust, these melts reach the surface or cool down during their ascent to such an extent that they solidify and form dikes or sills. A special form arises when curved strike-slip faults in crustal transtension zones have large offsets. In this case, grabens or rectangular pull-apart basins may form. Transport of magma with simultaneous extension of the crust hinders the ascent into higher sections, as the volume of the magma compensates for the space that is created. Depending on the dimensions involved, this prevents subsiding of the surface.
At the same time, a complex magma system arises in the crust with branched channels and pockets in which differentiation processes take place (Annen et al., 2006). Additionally, magma mixing takes place through repeated intrusions (Annen, 2011). Due to temperature differences between host and guest melts a special form of mixing with formation of melt droplets can occur in which diffusion processes form enriched "extraction" melts, e.g. phonolithic magmas (Schreiber et al., 1999).
During its ascent, the magma pushes a front of fluids along its top which are composed of supercritical phases of water and gases (scH2O, scCO2 and scN2) from the mantle and the crust. At the top in the uppermost area of the magma column, a kind of foam forms from the melt and fluids. Supercritical phases can be mixed indefinitely until a substance changes into another phase at lower pressure/temperature conditions.
For fluids, this first accounts for water, which is supercritical from 221 bar (at a depth of > 2.2 km of open water column) and a temperature of more than 374 °C. If one of the properties falls below these values, the transition to the liquid phase takes place. Such conditions are normally achieved in the deeper crust. For CO2, the transition from the supercritical state to the gas phase only occurs at a depth of approx. 750 m (based on an open water column) and/or when the temperature drops below the limit of 31 °C.
If magma rises in fault zones, it comes into contact with water in the crust. The temperature for the supercritical state of water is reached immediately. The pressure is sufficient for the supercritical state up to a depth of approx. 1 km (lithostatic pressure in a closed system). The question remains as to when the pressure falls below the limit value of 221 bar if ascent continues, so that the subcritical state is reached. Here, the ascent process of the magma in a feeder channel that has not yet or not yet sufficiently opened up to the surface of the earth must be taken into account.
The ascent, which is maintained by continuously intruding magma, is only made possible through the high pressure at the front of the magma column. In connection with the extremely low surface tension of supercritical phases at the top of the magma column, it ensures opening of fractures in fault zones and thus the formation of pathways which enable magma ascent.
The higher pressure at the top of the magma column compared to the surrounding rocks can thus ensure that the supercritical state of the fluids is maintained up to near the surface. Only in the uppermost part of the magma column the supercritical state is lost and a direct transition from the supercritical state to the water vapour and gas phase occur. The fact that more energy is stored in the supercritical phase than in a superheated vapour phase explains the high explosive power of the initial eruption. For example, deep-drilled supercritical water for geothermal power plants delivers 10-20 times more yield than traditional geothermal systems (Stober and Bucher, 2020).
Due to expansion of gases and water vapour, the temperature decreases significantly (Joule-Thomson effect) which quenches lumps of melt (unpublished discussion basis by co-author Schreiber). Depending on the location and the morphological situation, contact with groundwater and surface water can lead to steam-controlled (phreatic and phreatomagmatic) eruptions, which expand the primarily formed eruption funnels to the depth (Lorenz, 1973; Lorenz and Büchel, 1980 a; Büchel and Mertes, 1982).
The threat posed by maar volcanism is manageable. The eruptions are characterized by the ballistic transport of blocks of the host rock in the immediate vicinity of the eruption point as well as by lapilli and ash falls beyond this area. Since the volume of ashes released into the atmosphere is small, there is no danger at a safety distance of 10 km that roofs are overloaded or people and animals are directly endangered. Only thin tephra deposits are to be expected at a greater distance, depending on the prevailing wind direction.
According to Zolitschka et al. (1995), the thickness of the Ulmen Maar tephra from the youngest maar of the Eifel thins out from 15 m directly along the crater rim to 0.2 mm at the Meerfelder Maar at a distance of 21 km. Regional air traffic may be effected because ash eruptions, especially in connection with phreatomagmatic processes, can lead to an input into higher atmospheric strata.
Literatur
Annen, C. (2011): Implications of incremental emplacement of magma bodies for magma differentiation, thermal aureole dimensions and plutonism–volcanism relationships. Tectonophysics, 500, 3–10.
Annen, C., Blundy, J.D., Sparks, R.S. (2006): The genesis of intermediate and silicic magmas in deep crustal hot zones. Journal of Petrology, 47, 505–539.
Büchel, G., Mertes, H. (1982): Die Eruptionszentren des Westeifeler Vulkanfeldes. Z. dt. geol. Ges., 133, 409-429.
Lorenz, V. (1973): On the formation of maars. Bull. Volcanol., 37, 183-204.
Lorenz, V., Büchel, G. (1980a): Zur Vulkanologie der Maare und Schlackenkegel der Westeifel. Mitt. Pollichia, 68, 29-100.
Schreiber, U., Anders, D., Koppen, J. (1999): Mixing and chemical interdiffusion of trachytic and latitic magma in a subvolcanic complex of the Tertiary Westerwald (Germany). Lithos, 46, 695-714.
Stober, I. and Bucher, K.: Geothermie, Springer, Spektrum, 3rd Ed., 2020.
Zolitschka, B., Negendank, J.F.W., Lottermoser, B.G. (1995): Sedimentological proof and dating of the early Holocene volcanic eruption of Ulmener Maar (Vulkaneifel, Germany). Geol.Rundsch. 84, 1, 213–219.
9.1.4 Calderas
The discharge of magma chamber systems leads to the formation of collaps calderas, which give an initial indication of the magnitude of the magma volume involved. One of the oldest calderas of the East Eifel is interpreted to be located between Spessart and Kempenich (volcanological map of the East Eifel, vd Bogaard and Schmincke, 1990c; Schmincke, 2007). There are also newer indications from engineering geological investigations that corroborate the assumption (oral communication Dipl. Geol. A. Justen, Ing-Büro Wasser und Boden Boppart; Justen et al., 2004).
Near Rieden, there is a complex caldera structure that has evolved due to repeated volcanic activities over a period of almost 200 ka. Subsequently, a few kilometres further northeast near Wehr, another caldera developed as a result of at least two eruption cycles. The time interval between these eruptions is approx. 60 ka (Schmincke, 2007). Today, there is still strong CO2 degassing at various points in the caldera, some of which is used economically.
The most recent caldera formation in the Eastern Eifel took place around 13 ka (12,900 ± 560 a bp, vd Bogaard, 1995) due to the eruption of the Laacher See volcano. The basanitic/tephritic volcanism postulated by Förster et al. (2019) to have occurred at 24.3 ka at the location of today's Laacher See, from which the Eltville Tephra is said to have descended, would have been obscured by the subsequent Laacher See volcanic eruption about 13 ka ago.
All activities that led to a caldera in the East Eifel share a large-volume eruption process that far exceeds the erupted mass of the basaltic volcanic rocks. However, the calculations for the erupted volume of the Laacher See volcano, which is given as 6.3 km³, have to be checked. Neither terrain findings nor the caldera size of only half a cubic kilometre nor geophysical investigations suggest such volumes (see below).
9.3.1 Laacher-See volcano
The Laacher-See volcano (LSV) is the youngest in the East Eifel with an age of almost 13 ka (vd Bogaard, 1995; Litt et al., 2003; Schmincke, 2007). This means that weathering and erosion of the volcano are the least advanced. The good state of preservation of the tephra is one reason why the LSV could be examined in great detail. The thicknesses of the tephra were used as a basis to constrain the volume of the magma system (Diss. vd Bogaard, 1983).
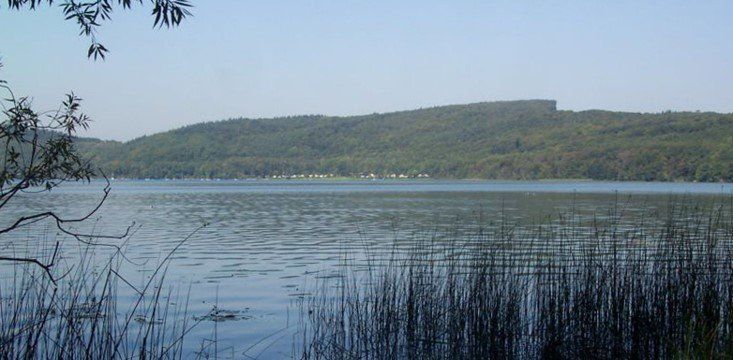
The erupted volume was initially given as 5.3 km³, after several revisions it was more than 6.3 km³ plus overlying wallrock of 0.5 km³ (vd Bogaard, 1983; Wörner and Schmincke, 1984 a, b; Wörner et al., 1985; Wörner and Wright, 1984; vd Bogaard and Schmincke, 1985, Freundt and Schmincke, 1986; Harms and Schmincke, 2000; Harms et al., 2004; Schmincke, 2007). When the melt is foamed up into pumice, the volume increases by about three times during the eruption.
The calculation of the tephra thicknesses in the proximal and distal area of the LSV resulted in approx. 18 km³. From this, the volume of the phonolithic magma with 6.3 km³ to a maximum of 6.5 km³ (DRE, Dense Rock Equivalent) was calculated, and the volume of the basanitic initial magma was determined. This provided a basis for calculating the order of magnitude of the other highly differentiated volcanoes Wehr, Rieden (4 km³), Brenk and others.
After all assumptions and calculations, the volume of the entire erupted magma in the West and East Eifel should be 20 km³ or possibly 30 km³. The initial volume from which the differentiated magmas developed in the crust is estimated at a size of 200-300 km³ for the volcanoes of the East Eifel (Schmincke, 2007).
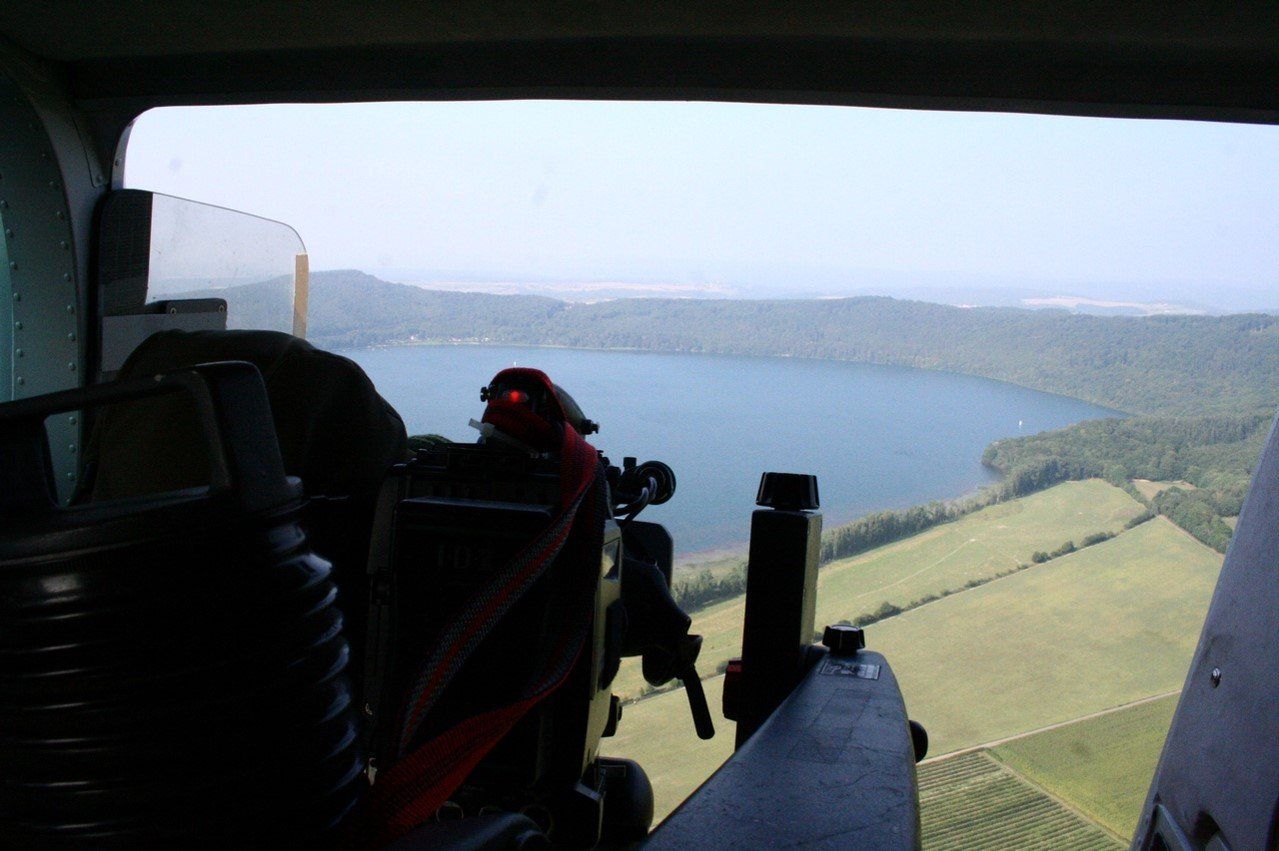
Schmitt et al. (2010) used U-Th dating of intrusive carbonatites to propose the existence or accumulation of the developed Laacher-See magma system at least 20 ka before the phonolite eruption. Since cooling and differentiation in a magma system take place parallel to the emplacement, the entire process of chamber formation of the LSV occurs within a few tens of thousands of years (see also Annen, 2011; Annen et al., 2006).
From this, a problem exists that has not yet been explained. The magma chamber of the LSV is said to have been located at a depth of 4.6–7.8 km (Harms et al., 2004), i.e. the brittle upper crust, which can only be opened by movement along tectonic faults. The volume of 6.3 km³ plus the amount that remained in the magma chamber as residue requires a base area of at least 1.5 km x 1.5 km for a cuboid with a height of around 3 km. The initial volume is said to have been a basanitic magma with 16.6 km³, which was also located in the upper crust (vd Bogaard and Schmincke, 1984), of which 11 km³, in an alternative approach 50 km³ (Wörner and Schmincke, 1984a) remained in the middle crust.
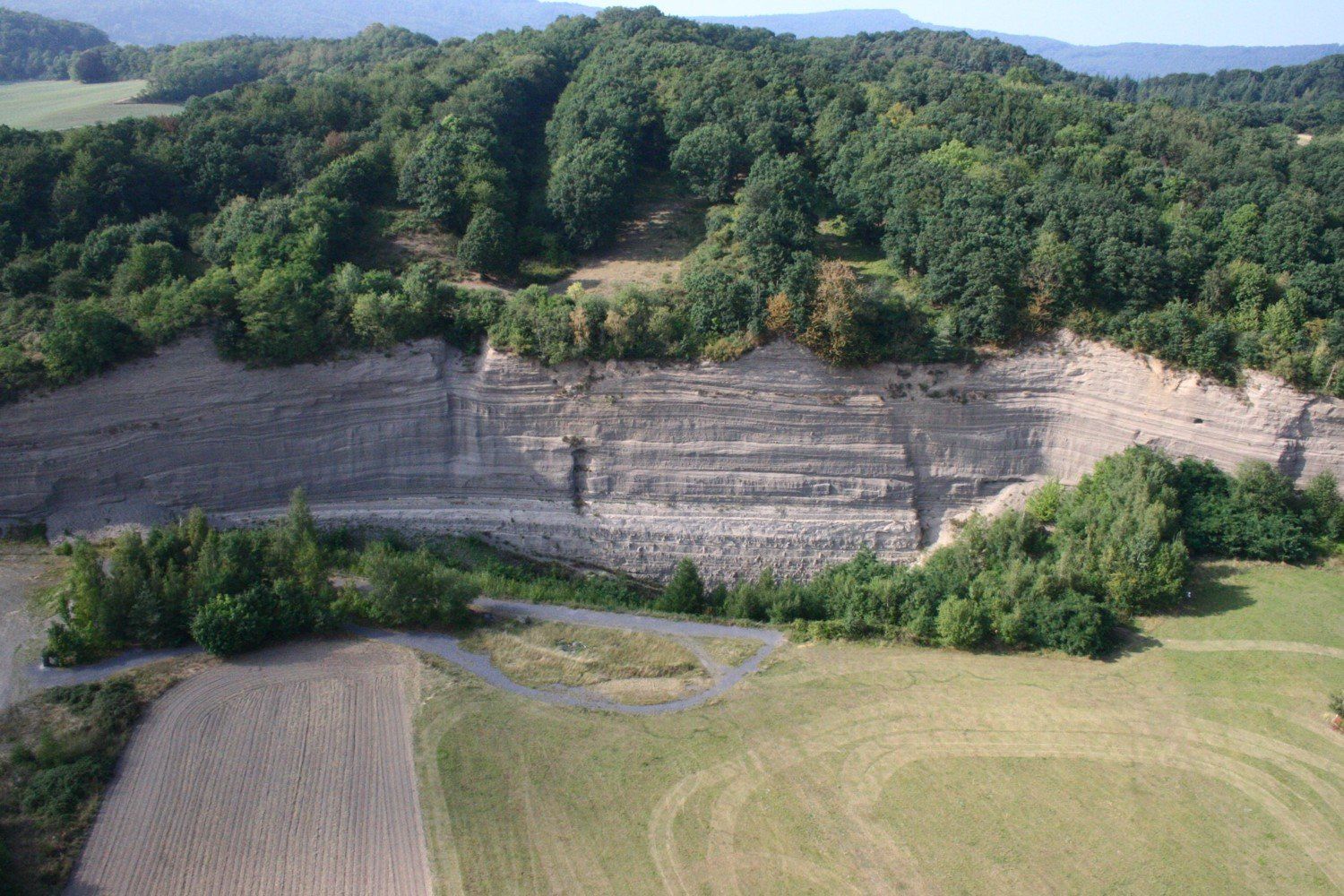
For the opening of at least 6.3 km³ of potential storage space, a part of the crust must be pulled to the side for 1.5 km at depth (under the above conditions) in order to achieve the corresponding volume. For a formation period of 20 ka (according to Schmitt et al., 2010), the velocity would be in the order of 75 mm/year. The recent movement rates in fault zones in the Eifel are, according to the evaluation of fault plane solutions, in the order of magnitude of 0.06 to 1.7 mm/year (Demoulin, 2004; Demoulin et al., 2005; Hinzen, personal communication). The uplift rates are 0.35 mm/year, with a maximum uplift of 3.5 mm/year (Garcia-Castellanos et al., 2000; Meyer and Stets, 2002; Mälzer et al., 1983; Campbell et al., 2002) or for large parts of the Eifel according to the latest studies at 1 mm per year (Kreemer et al., 2020).
So far it has not been explained tectonically how the build-up of the magma chamber could have taken place on the scale described and in the postulated period of time. Geophysical investigations in the vicinity of the Laacher See have so far not produced any evidence of structures that correspond to a relict magma chamber of 11 km³ or 50 km³ (Ahorner, 1983, magnetotellurics; Lohr, 1982, gravimetry; Ahorner et al., 1986; Köhler, 2005, low velocity studies; Ochmann, 1988, seismology; Pucher, 1992, magnetics).
The DEKORP research group (1991), after evaluating the profile on the northern edge of the Laacher See, came to the conclusion that there is no relict magma chamber with a diameter of more than 3 km at a depth of less than 8 km. The remaining residual magma should, however, be clearly measurable.
The caldera size of the LSV provides hints to a misjudgement of the magma chamber volume. It has a volume of half a cubic kilometre (Viereck and vd Bogaard, 1986). The cavity created by the collapse of the crustal roof roughly represents the erupted volume, as there was no volcanic cone that was destroyed with the eruption and integrated into the Tephra (Schmincke, 2009).
It has not been clarified how the volume difference of 6 km³ between the caldera and the postulated amount of tephra (plus adjacent rock of the overburden) is balanced. A cavity at this depth from this volume is completely excluded, just like the consideration by Viereck and vd Bogaard (1986) that subsequent magma (6 km³ within a few days) could have compensated for the subsidence of the caldera (there is no geophysical evidence for this calculation).
Compared to published model studies, the dimensions of the Laacher-See magma chamber can be reduced to 10 to 20 percent of the size previously postulated. Ochmann (1988, Fig. 9.7) gives an alternative interpretation for the magma chamber system of LSV.
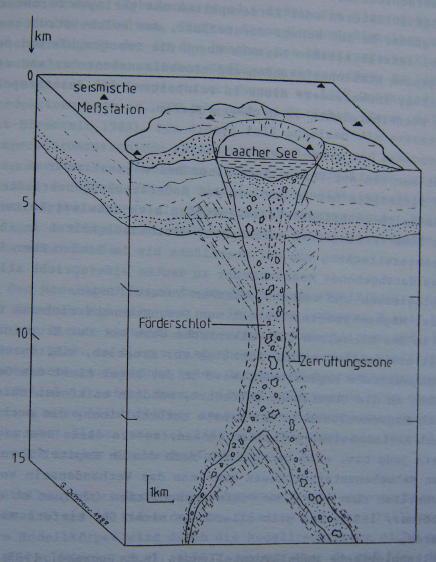
Fig. 9.7 from Ochmann, (Diss. 1988, Fig. 6.17): Strong mechanical fracturing in the area of the vent of the Laacher See volcano. Hypothetical interpretation of a tomographic structure analysis based on teleseismic studies. The model shows two vents of different dimensions at a depth of more than 10 km.
The model concepts outlined above for the volume of the involved magmas in the entire Eifel, which are causally related to the mantle plume, are extrapolated from the assumptions about the volume of the Laacher-See magma chamber (Schmincke 2007). If only 10 to 20% of the postulated values are used for this, the total volume of the two quaternary volcanic fields is reduced accordingly.
In order to get clarity on this, a review of the raw data and models that were used as the basis for the calculation of the Laacher-See magma chamber would be necessary. It would also be essential to integrate the tectonics in connection with the previously carried out geophysical investigations as well as a modelling of the eruption processes using modern volcanological methods.
Atmospheric flow models (unequal wind displacement during the eruptions, dune formations, dust storms long after the tephra has been deposited) and, for the proximal fallout areas, slope wash-offs and alluvial fan effects should be taken into account. Since the LSV eruption was the largest eruption in the Eifel to date, the information and data about its primary and secondary effects should be taken into account when discussing scenarios and locations for radioactive waste repositories.
Literature
Ahorner, L. (1983): Historical seismicity and present-day microearthquake activity of the Rhenish Massif, Central Europe. In: Fuchs, K., Von Gehlen, K., Mälzer, M., Murawski, H., Semmel, A. (Eds.), Plateau Uplift, The Rhenish Shield—A Case History. 198– 221; Berlin (Springer).
Ahorner, L., Budny, M., Ochmann, N., Wohlenberg, J. (1986): Integrated seismic prospection of a potential geothermal deposit in the volcanic area of the western Eifel. Final report. P 191 Integrierte seismologische Prospektion einer möglichen geothermischen Wärmelagerstätte im Vulkangebiet der Westeifel. Schlussbericht DE-88-012035; EDB-88-183263.
Annen, C. (2011): Implications of incremental emplacement of magma bodies for magma differentiation, thermal aureole dimensions and plutonism–volcanism relationships. Tectonophysics, 500, 3–10.
Annen, C., Blundy, J.D., Sparks, R.S. (2006): The genesis of intermediate and silicic magmas in deep crustal hot zones. Journal of Petrology, 47, 505–539.
Bogaard, vd P. (1983): Die Eruption des Laacher See Vulkans. Diss. Ruhr Univ. Bochum. 340 S.
Bogaard, vd P. (1995): 40 Ar/39 Ar ages of sanidine phenocrysts from Laacher See Tephra (12,900 years BP): chronostratigraphic and petrological significance. Earth Planet. Sci. Lett. 133, 163-174.
Bogaard, vd P., Schmincke, H.-U. (1984): The eruptive center of the late Quaternary Laacher See Tephra. Geol. Rdsch. 73, 935–982.
Bogaard, vd P., Schmincke H.-U. (1985): Laacher See Tephra: a widespread isochronous late Quaternary tephra layer in central and Northern Europe. Geol. Soc. Am. Bull. 96, 1554–1571.
Bogaard, vd P., Schmincke H.-U. (1990c): Vulkanologische Karte der Osteifel, 1:25.000, Pluto Press, Witten/Kiel.
Campbell, J., Kümpel, H.-J., Fabian, M., Fischer, D., Görres, B., Keysers, C.J., Lehmann, K. (2002): Recent movement pattern of the Lower Rhine Basin from tilt, gravity and GPS data. Neth. J Geosc. /Geologie en Mijnbouw, 81,2, 223–230.
DEKORP Research Group (1994): The deep reflektion seismic profiles DEKORP 3 / MVE-90. Zeitschrift für Geol. Wissenschaften, 22, 6, 624 – 824.
Demoulin, A. (2004): Reconciling geodetic and geological rates of vertical crustal motion in intraplate regions. Earth and Planetary Science Letters, 221, 91-101.
Demoulin, A., Campbell, A., Wulf, A. De., Muls, A, Arnould, R., Görres, B., Fischer, D., Kötter, T., Brondeel, M., Damme, D. van, Jacqmotte, J.M. (2005): GPS monitoring of vertical ground motion in northern Ardenne–Eifel: five campaigns (1999–2003) of the HARD project. Int. J. Earth. Sci. (Geol. Rundsch.) 94: 515–524. doi 10.1007/s00531-004-0450-3
Förster, M.W., Zemlitskaya, A., Otter, L.M., Buhre, S., Sirocko, F. (2019): Late Pleistocene Eifel eruptions: insights from clinopyroxene and glass geochemistry of tephra layers from Eifel Laminated Sediment Archive sediment cores. J. Quaternary Sci., 35, 186-198. doi:10.1002/jqs.3134
Freundt, A., Schmincke, H.-U. (1986): Emplacement of small-volume pyroclastic flows at Laacher See (East-Eifel, Germany). Bull. Volcanol. 48, 39-59.
Garcia-Castellanos, D., Cloetingh, S., Balen, R. van (2000): Modelling the middle Pleistocene uplift in the Ardennes-Rhenish Massif: thermo-mechanical weakening under the Eifel. Global Planet. Changes, 27: 39–52.
Harms, E., Schmincke, H.-U. (2000): Volatile composition of the Laacher See Phonolite magma (12 900 yr BP): implications for syneruptive degassing of S, F, Cl, H2O. Contributions to Mineralogy and Petrology., 138, 84–98.
Harms, E., Gardner, J.E., Schmincke, H.-U. (2004): Phase equilibria oft he Laacher See Tephra (East Eifel, Germany): constraints on pre-eruptive storage conditions of a phonolithic magma reservoir. J. Volcanol. Geotherm. Res., 134, 125-138.
Justen, A., Köppen, K.-H., Dreher, T. (2004): Kohlensäureführende Grundwässer in der Caldera von Weibern-Rieden, Osteifel. Symposium der International Association of Hydrogeologists, Andernach 25. bis 26. Nov.
Köhler, C. (2005): Gravimetrische Untersuchungen am Südrand des Laacher Sees zur Auflösung der Untergrundstruktur im Randbereich des Vulkans. Diplomarbeit, Technische Universität Bergakademie Freiberg, Institut für Geologie.pp.77.
Kreemer, C., Blewitt, G., Davis, P.M. (2020): Geodetic evidence for a buoyant Mantle Plume beneath the Eifel volcanic area, NWEurope. Geophys. J. Int., 222, 1316–1332.
Litt, T., Schmincke, H.-U., Kromer, B. (2003): Environmental response to climate and volcanic events in central Europe during the Weichselian Lateglacial. Quaternary Science Reviews 22, 7–32.
Lohr, U. (1982): Ergebnisse magnetotellurischer Messungen im Gebiet des Laacher Sees. – Diss. TU-Braunschweig, 95 Seiten, Braunschweig.
Mälzer, H., Hein, G., Zippelt, K. (1983): Height changes in the Rhenish Massif: determination and analysis. In: Fuchs, K., Von Gehlen, K., Mälzer, M., Murawski, H., Semmel, A. (Eds.), Plateau Uplift, The Rhenish Shield— A Case History., 165–176. Berlin (Springer).
Meyer, W., Stets, J. (2002): Pleistocene to Recent tectonics in the Rhenish Massif (Germany). Neth. J. Geosci., 81, 217– 221.
Ochmann, N. (1988): Tomographische Analyse der Krustenstruktur unter dem Laacher See Vulkan mit Hilfe von teleseismischen Laufzeitresiduen. Diss. RWTH Aachen, 105 S., Aachen.
Pucher, R. (1992): Magnetfeldanomalien in der Eifel. Geol. Rundsch., 81, 429 -443.
Schmincke, H.-U. (2007): The Quaternary Volcanic Fields of the East and West Eifel (Germany) In: Ritter, R., Christensen, U. (Eds.) (2007): Mantle Plumes – A Multidisciplinary Approach. 241-322.
Schmincke, H.-U. (2009): Vulkane der Eifel. Spektrum, Heidelberg.
Schmitt, A.K., Wetzel, F., Cooper, K.M., Zou, H., Wörner, G. (2010): Magmatic longevity of Laacher See Volcano (Eifel, Germany) indicated by U–Th dating of intrusive carbonatites. Journal of Petrology, 51, 1053–1085.
Viereck, L.G., Bogaard, vd P. (1986): Magma‐ und Wärmeinhalt der Magmakammer des Laacher See und des Riedener Vulkans. Forschungsbericht T 86‐174. Bundesministerium für Forschung und Technologie (BMFT).
Wörner, G., Schmincke, H.-U. (1984a): Mineralogical and chemical zonation of the Laacher See Tephra Sequence (East Eifel, W. Germany). J Petrol., 25, 805–835.
Wörner, G., Schmincke, H.-U. (1984b): Petrogenesis of the zoned Laacher See Tephra. J. Petrol., 25, 836–851.
Wörner, G., Wright, T.L. (1984): Evidence for magma mixing within the Laacher See magma chamber (East Eifel, Germany). J. Volcan. Geotherm. Research, 22, 301–327.
Wörner, G., Staudigel, H., Zindler, A. (1985): Isotopic constraints on open system evolution of the Laacher See magma chamber (Eifel, West Germany). Earth and Planetary Science Letters, 75, 37–49.